Advances in Morphodynamic Modeling of Coastal Barriers: A Review
Publication: Journal of Waterway, Port, Coastal, and Ocean Engineering
Volume 149, Issue 5
Abstract
As scientific understanding of barrier morphodynamics has improved, so has the ability to reproduce observed phenomena and predict future barrier states using mathematical models. To use existing models effectively and improve them, it is important to understand the current state of morphodynamic modeling and the progress that has been made in the field. This manuscript offers a review of the literature regarding advancements in morphodynamic modeling of coastal barrier systems and summarizes current modeling abilities and limitations. Broadly, this review covers both event-scale and long-term morphodynamics. Each of these sections begins with an overview of commonly modeled phenomena and processes, followed by a review of modeling developments. After summarizing the advancements toward the stated modeling goals, we identify research gaps and suggestions for future research under the broad categories of improving our abilities to acquire and access data, furthering our scientific understanding of relevant processes, and advancing our modeling frameworks and approaches.
Introduction
Coastal barriers are narrow landforms that are separated from the continental mainland by a shallow waterbody (Fig. 1). These barriers can be book-ended by inlets (i.e., barrier islands) or they can be connected to the mainland at one end (i.e., barrier spits) or both (baymouth barriers). The combination of backbarrier environment, subaerial island, and shoreface are often succinctly referred to as the “barrier system” or simply “barrier.” As of 2011, over 20,000 kilometers of the world’s coasts were characterized by a barrier system, accounting for approximately 10% of all coastlines (Stutz and Pilkey 2011). Barriers provide significant benefits during coastal storms such as surge volume and wave energy reduction (Grzegorzewski et al. 2011), wetland protection (Wamsley et al. 2009), sediment stabilization through the presence of subaerial or backbarrier vegetation, and protection of aquatic habitat (Bridges et al. 2013). In addition, barrier islands have become popular as both vacation destinations (Pilkey et al. 2011) and permanent residential areas, which has led to increases in population density (Zhang and Leatherman 2011).

Fig. 1. Satellite and aerial images of a Virginia Barrier Island: (a) location map; (b) Delmarva Peninsula (base map from ESA 2021); (c) Wallops Island; and (d) zoomed section of Wallops Island.
[Base maps (b) and (c) from VGIN 2021.]
Although many barriers have undergone rapid urban development since the mid-20th century (Dolan and Lins 1986), Stutz and Pilkey (2011) described this development boom as being “ironically” timed due to coastal hazard accelerations associated with current trends in sea level rise (SLR). According to the Intergovernmental Panel on Climate Change, global mean sea level (MSL) is predicted to rise between 0.25 and 1.0 m by the end of the century (Oppenheimer et al. 2019). If these predictions hold true, the rates of barrier island morphological change and associated flooding during storms and other events will most certainly increase (e.g., Gutierrez et al. 2007). In addition to exacerbating coastal flooding, SLR also drives the evolution of the barrier system itself, influencing processes that change both the island’s shape and location. Thus, on many barrier coastlines, permanent structures have been constructed on land that was and is expected to continue migrating toward the mainland (Pilkey et al. 2011). Changes in the location and geometrical configuration of barrier systems are expected to alter the benefits that they provide to neighboring mainland communities. Therefore, it is critically important for all who are involved in coastal management to understand barrier island morphodynamics to produce the best possible outcomes for coastal communities.
While the earliest literature tended to document observations and initial theories of barrier morphodynamics, research has recently—in the last three or so decades—shifted toward the development and intensified use of computational models. Based on this observation, we note that where modeling often lagged behind or paralleled our advancements in scientific understanding, it has recently been used to validate and advance it. Many models have been developed over the last 3–4 decades. A review of these models may help new or future researchers survey the field of barrier morphodynamic modeling.
A few notable review papers have recently been published related to barrier morphodynamics. Some of these papers focus on a single, specific component of coastal change such as overwash (e.g., Donnelly et al. 2006) or storm sequencing and recovery (e.g., Eichentopf et al. 2019). Other reviews capture the larger-scale morphological response of barrier systems, but their application is either constrained to a particular location (e.g., Rosati and Stone 2009), focused on a particular driver such as climate change (e.g., Toimil et al. 2020), or focused in-depth on a particular spatiotemporal scale (e.g., Sherwood et al. 2022). Table 1 provides a summary of these reviews and their focus areas. These reviews provide a valuable synthesis of relevant work but are not sufficient to capture the trends and advancements in barrier morphodynamic modeling.
Citation | Focus |
---|---|
Donnelly et al. (2006) | Laboratory work, field studies, and modeling efforts related to coastal overwash. |
Rosati and Stone (2009) | Barrier evolution concepts from early literature; recent concepts in Northern Gulf of Mexico. |
McBride et al. (2013) | Observations and conceptual models of barrier morphodynamics for various coastlines and regional locations. |
Chardón-Maldonado et al. (2016) | Recent advancements on hydrodynamics and sediment transport modeling in the swash zone. |
Reeve et al. (2016) | Long-term morphodynamic models that employ data-driven and/or hybrid approaches. |
Ciavola and Coco (2017) | Event-scale processes and their impact on specific coasts (e.g., sandy beaches, barrier islands, tidal flats, etc.). |
Moore and Murray (2018) | Compilation of recent work and synthesis of current understanding and state of research on barrier morphodynamics. |
Eichentopf et al. (2019) | Laboratory studies, field work, and modeling exercises related to storm sequencing and beach recovery. |
Ranasinghe (2020) | Commonly used morphodynamic models for sandy beaches and ideas for future long-term models. |
Toimil et al. (2020) | Coastal erosion modeling, climate change impacts, and approaches for evaluating uncertainty. |
Sherwood et al. (2022) | Advances in modeling event-driven morphodynamics on sandy coasts. |
The purpose of this manuscript is to fill that gap by providing a review of the literature regarding advancements in morphodynamic modeling of coastal barrier systems. Our review of modeling advancements is divided in two broad categories: (1) event-scale morphodynamics, and (2) long-term morphodynamics - refer to the “Terminology” section for definitions of “event-scale” and “long-term.” These sections begin with a brief description of commonly modeled phenomena and processes, followed by a review of relevant modeling efforts, which are categorized according to their primary intent. At the conclusion of these sections, we summarize the primary contributions of the modeling developments and their limitations. Finally, we conclude with the identification of research gaps that currently exist and suggest directions for future research.
A few items should be noted regarding this study. First, there are some relevant topics (e.g., anthropogenic impacts, influences of vegetation) which are only briefly discussed due to our focus on morphodynamic modeling. Second, we have intentionally included many models and/or modeling approaches from the early literature so that the current models might be understood in their proper historical context, which requires knowledge of both previous and ongoing efforts. In addition, this review primarily focuses on models in wide use in the research community. Therefore, some commonly used propriety models have only been briefly mentioned. Lastly, although our review is focused on barrier morphodynamics, many of the relevant processes play an important role for nonbarrier coasts. Therefore, to fully understand the modeling advancements relevant to barrier systems, we must consider some modeling efforts that are not barrier-specific.
Before starting this review, it may be helpful to orient the unfamiliar reader by defining our modeling goals and our terms. In the next section, we have attempted to summarize our modeling goals with one overarching statement or Grand Challenge. This is followed by a brief discussion of terminology used in this manuscript.
Grand Challenge
In theory, the ideal morphodynamic model would produce accurate predictions in a reasonable time without significant computational expense. As we consider how these ideals translate into reality, there are multiple modeling goals that we must work toward and important intermediate steps that we must first achieve. However, rather than outlining each goal, we have attempted to synthesize them into a single overarching goal, or Grand Challenge, as follows:
To predict barrier system morphodynamics in multiple spatiotemporal dimensions (e.g., short to long time scales, transect to regional evolution) with a high degree of confidence, under reasonable computational resources constraints, and considering relevant factors such as event-driven morphological change, evolution during nonstormy periods, biological processes (and other potential subsystem influences), and anthropogenic impacts.
We intend the phrase “predict… with a high degree of confidence” to mean predictions that have at least been partially validated and are useful in planning and decision-making. Throughout this review, the reader is encouraged to consider each development in light of the Grand Challenge. At the conclusion of each major section, we summarize the modeling advancements and extant limitations, offering our perspectives on progress toward this overarching goal. To maintain this focus, it should be noted that some relevant topics such as biological processes and anthropogenic impacts are given more of a cursory discussion.
Terminology
There are some inconsistencies in terminology in the body of work on barrier morphodynamics. Thus for the purpose of this review, our aim here is to define terms that describe what is being modeled (e.g., a phenomenon, a process), the types of mathematical representations that are used (e.g., a model, a formulation), and the spatiotemporal scales used throughout the paper.
When discussing phenomena, we are talking about observable characteristics, behaviors, or events of a system. While the spatiotemporal scales of a system may vary (e.g., initiation of particle movement versus shoreline behavior), there are phenomena associated with each system that may be mathematically represented via the development of a model. When we discuss processes, we are referring to patterned events that systematically contribute to the observable phenomena of a system. Based on these terms, we also distinguish between models and formulations. Whereas models are developed to represent phenomena, specific formulations are developed to represent processes. Models, therefore, may contain one or more formulations of a process. For example, consider the development and growth of a spit. The spit development and/or growth would be the observed phenomenon that is systematically progressed by the process of longshore sediment transport (LST). Thus, we might develop a model that predicts spit development and growth using a specific LST formulation.
The last terms that need to be defined up front are related to the spatial and temporal scales at which the relevant processes are typically resolved in coastal morphodynamic modeling. Herein, we adopt the temporal scale classification of Rosati and Stone (2009), and adopt a slightly modified version of the spatial scale classification of Cowell et al. (2003a). These scales are presented in Table 2 and are used throughout this paper. Note, we also use the term “event scale” throughout this manuscript to refer to the combination of small spatial and short temporal scales.
Type | Term | Scale |
---|---|---|
Spatial | Small-scale | 100–102 m |
Spatial | Moderate-scale | 102–103 m |
Spatial | Large-scale | >103 m |
Temporal | Short-term | Hours to days |
Temporal | Mid-term | Days to decades |
Temporal | Long-term | Decades to centuries |
Event-Scale Morphodynamics
This section provides an overview of commonly modeled phenomena and processes associated with event-scale morphodynamics, a review of relevant modeling efforts, and a summary of advancements toward the Grand Challenge.
Commonly Modeled Phenomena and Processes
Acute sediment transport processes, which are characterized by a sudden onset and short-term duration, are initiated when a storm approaches the coast. Chronic transport processes, which are characterized by gradual beginnings and mid- to long-term duration, are not initiated during storms but are intensified by them. As these transport processes are initiated or intensified, the barrier responds in the form of morphological adjustment. To frame the discussion on storm response, we use the storm impact scale published by Sallenger (2000), wherein acute processes occur within four regime classifications: swash, collision, overwash, and inundation (Fig. 2). Each regime has certain morphological responses associated with runup levels.

Fig. 2. Storm impact scale.
(Adapted from Sallenger 2000.)
In the swash and collision regimes, increased water levels by storm surge and wave runup lead to increased erosion on the beach and dune, depositing the eroded material seaward of the beach. Collision differs from swash in that the water level exceeds the dune toe, allowing waves to collide with and erode the lower parts of the dune slope, which can lead to avalanching of the upper dune. Sallenger (2000) points out that while sediment transported offshore under this regime may return to the beach, this sediment typically does not make it back to the dune structure, resulting in net erosion of the dunes over time. In the overwash regime, water levels are high enough such that incident wave runup intermittently flows over the dune peaks or antecedent low spots, carrying mobilized sediment with it. Lastly, the inundation regime involves complete submergence of the barrier which can lead to inlet formation (i.e., breaching) and significant increases in the cross-shore sediment transport (XST) rates (Sallenger 2000). Inundation is associated with extreme levels of erosion that pick up normally dry (subaerial) sediment.
One regime that Sallenger does not include is the outwash regime, following the terminology proposed by Over et al. (2021), which describes seaward flows and associated offshore sediment transport. Although it is possible to have initial seaward surge depending on the orientation of the islands and the approach angle of the storm, initial surge levels are typically directed onshore. Therefore, seaward flows associated with the outwash regime usually occur after storms make landfall or pass by the area of interest, which reverses the predominant wind direction. Applied to a typical barrier system, this reversed wind field can cause backbarrier water levels to surge above receding ocean-side water levels. In this instance, breaching may be initiated from the backbarrier by outwash flows that scour a new channel through the island, liquefaction of previously weakened dune structures, or a combination of both. Various studies including Shin (1996), McCall et al. (2010), Smallegan and Irish (2017), Harter and Figlus (2017), and Over et al. (2021) highlight the importance of considering this regime when modeling storm event morphodynamics.
The following sections offer an introductory discussion on commonly modeled phenomena and processes associated with barrier response to storm events, namely profile erosion and shoreface response, overwash, and breaching. This is followed by a review of relevant modeling efforts.
Beach Profile Erosion and Shoreface Response
While the term “profile” can be used to describe a wide range of the barrier system, we use the term “beach profile” herein to describe the morphodynamic response of the barrier’s beach–dune complex and upper shoreface, which we loosely define as the morphologically “active zone” following Stive and de Vriend (1995) and Cowell et al. (2003a). Generally, there are two primary factors that contribute to erosion of the beach profile under storm conditions: (1) increased offshore-directed currents; and (2) increased total runup. As the waves intensify, the beach profile state turns erosional (assuming a prior accretive state) as wave-driven sediment transport becomes dominated by undertow and rip currents which are offshore-directed (Aagaard and Kroon 2017). Sediment is eroded from the upper portions of the profile and deposited on the shoreface, typically in a subaqueous bar, which is then delivered back to the profile once storm conditions subside (Quartel et al. 2007). This cycle of erosion and subsequent recovery has been observed over seasonal wave-climate changes (Shephard 1950) and event-scale changes (Ranasinghe et al. 2012b). Second, the total runup, as produced by a combination of storm surge, astronomical tide, and wave runup, may exceed the swash regime water level to collide with the dune and cause notching (i.e., erosion and recession of the lower dune), followed by slumping or avalanching (Edelman 1968; Roelvink et al. 2009). For a more thorough review of sediment transport processes during storms and relevant factors, including the role of infragravity waves and incident wave nonlinearity, the reader is referred to Aagaard and Kroon (2017) and references therein.
These two primary factors (i.e., increased offshore-directed currents and increased total runup) contribute to barrier morphodynamics in significant ways. For example, in the collision regime, they lead to a net loss of sediment offshore to the lower (inactive) profile (Sallenger 2000). This net loss effectively limits the ability of the beach and dunes to fully recover to prestorm conditions without requiring external sediment sources (i.e., from the shelf, erodable profile outcrops, or LST gradients). Moreover, although much of the eroded sediment is brought back to the beach and dunes after the storm, this natural renourishment of the profile is not instantaneous, but can take days or weeks to recover (e.g., List et al. 2006; Quartel et al. 2007), leaving the barrier system in a temporarily hypervulnerable state. Profile recovery between storm events, although less studied than erosional events, is critically important to understanding barrier vulnerabilities to storm sequences and long-term morphology (Eichentopf et al. 2019).
Overwash
Overwash occurs when water flows over the dunes. Sediment is carried by the water and deposited behind the dunes as washover. While overwash was associated with intermittent overtopping in Sallenger’s overwash regime, it should be noted that by definition, overwash also occurs during Sallenger’s inundation regime and the proposed outwash regime, as the landward or seaward directed flows continue to transport sediment across the dunes. Donnelly et al. (2006) offered distinct definitions for runup overwash and inundation overwash and discussed the differences and implications of each process.
Three factors are the primary contributors to increased likelihood of barrier island overwash: (1) antecedent low spots in barrier topography; (2) high water levels driven primarily by storm surge; and (3) large incident waves. Although it can be argued that this is self-evidently true, it is also confirmed in the early literature on barrier island storm response (e.g., McCann 1979; Cleary and Hosier 1979). In addition to these three main contributing factors, overwash occurrence has also been associated with other variables including previous overwash activity, barrier island width, and vegetation density (McCann 1972; Fisher and Simpson 1979; Cleary and Hosier 1979). However, some of these factors can be indirectly related to antecedent topography. For example, areas that have experienced previous overwash events are also locations where the dunes have likely been lowered; thus, previous overwash activity can be linked to prestorm discontinuities in the dune elevation. Similarly, since dune vegetation promotes sediment settling and dune growth, vegetation density could generally be considered a proxy for prestorm topography. Donnelly et al. (2006) identified two other important factors including the direction of storm approach, which influences incident wave heights, and nearshore bathymetry, which impacts wave transformation.
Storms have significant morphological impact on barrier islands, which in turn affect the continued evolution and response to future storms. Observations from the early literature describe both destructive and constructive effects of overwash: destructive in that overwash may lower or destroy the dunes (e.g., Nichols and Marston 1939) and constructive in that overwash may contribute to aggradation of the barrier islands over time (e.g., Rosen 1979). Both of these effects directly impact flood risk from future storms. Again, to avoid duplicating work, the reader is referred to the review by Donnelly et al. (2006), which covers a variety of topics related to overwash including field and laboratory studies, modeling efforts, and its impact on barrier morphodynamics.
Breaching
Breaching is the creation of an inlet in a barrier that establishes direct hydraulic connectivity between the ocean and backbarrier water body (Kraus and Hayashi 2005). Breaches have been shown to account for water level increases both during the storm event in the form of bay surge (e.g., Cañizares and Irish 2008) and after the storm event in the form of increased tidal range (e.g., Conley 1999). Excess flooding, property damage, habitat loss, and decreased navigability are possible negative outcomes from a breach; however, breaching is also desirable in some cases and may be intentionally performed to increase habitat connectivity for certain estuarine wildlife (Gerwing et al. 2020) or to prevent undesirable backbarrier conditions including low salinity, poor water quality, and in some cases flooding (Kraus and Wamsley 2003).
From some of the earliest published observations of breaching, we know that multiple breaches, of various widths and depths, may form and expand during a single storm event (e.g., Nichols and Marston 1939). More recent studies have highlighted the dynamic nature of breaches, which can significantly change dimensions over relatively short time periods and even migrate alongshore (Kraus and Wamsley 2003; Wamsley and Kraus 2005). Timing of the initial breaching process has received relatively little attention in the literature due to the difficult nature of collecting field data. However, a study by Visser (1998) and a related modeling exercise by Roelvink et al. (2009) estimated lateral growth rates of breaches between 1 and 2 cm/s during initial formation. During the phases of breach growth, XST is much greater than LST; however, once flow in the breach ceases, LST may cause closure of the breach (Kraus et al. 2002).
In exploring the causes of breaching, researchers have often wanted to know on which side of the barrier breaching is initiated. Multiple theories of breach formation are present in the early literature, as reviewed by Pierce (1970), including breaching from the backbarrier side through the escape of impounded water (Shaler 1895) and ocean-side breaching by wave-driven erosion (Johnson 1919). Pierce (1970) determined that barriers are most likely to breach from the lagoon side but stated that a narrow barrier could also be breached by erosion from the sea. Although this perspective was published as early as 1970, it received little attention until recent years (e.g., McCall et al. 2010; Sherwood et al. 2014; Harter and Figlus 2017; Smallegan and Irish 2017).
Kraus et al. (2002) described two breaching processes and their association with lagoon-side or ocean-side breaching. The two processes are: (1) scouring and channelization and (2) seepage and liquefaction. Scouring and channelization most commonly occur from the seaward side of the barrier, when sustained storm surge allows for water to (semi)continuously inundate the island with flow over the barrier; conversely, seepage and liquefaction typically initiate breaching from the landward side of a narrow barrier (Kraus et al. 2002). However, recent modeling studies (e.g., Shin 1996; McCall et al. 2010; Sherwood et al. 2014; Smallegan and Irish 2017) have also shown that seaward-sloping water level gradients that occur after the ocean-side’s peak storm surge have the potential to scour channels across the barrier as well that can lead to seaward sediment transport and breaching.
Modeling Efforts
As stated previously, modeling efforts are classified according to their primary intent. Most event-scale morphodynamic models or formulations were developed to simulate a few key phenomena or processes including: (1) beach and dune erosion; (2) shoreface response; (3) overwash; (4) breaching; and (5) combinations of categories (1) through (4). The following sections review the relevant modeling efforts which are also graphically summarized in Fig. 3.

Modeling Beach and Dune Erosion
Modeling work on storm-driven response of the beach–dune complex was initiated and significantly advanced by researchers in the Netherlands in the 1960s and 70s. Edelman (1968) observed that when storm surge levels exceeded the dune toe, the dune would undergo significant erosion and partial avalanching. Based on these observations, Edelman published the first analytical formulation (i.e., method with a closed-form solution) for predicting dune erosion and retreat, later termed the “Provisional Method.” This method assumed the formation of a new dune toe at the peak storm surge elevation and balanced the volume of sediment eroded from the dunes with deposition in the nearshore zone [Fig. 4(b)] using linear approximations of both the nearshore and dune profiles. Four years later, Edelman used the same principles to publish a similar method which considered more realistic (e.g., nonlinear) profile shapes (Edelman 1972). In addition to sediment conservation and the new dune toe location, Edelman’s work was based on other key assumptions including a constant profile shape, rapid (or instantaneous) profile response, and the presence of both storm and pre/poststorm equilibrium profiles.
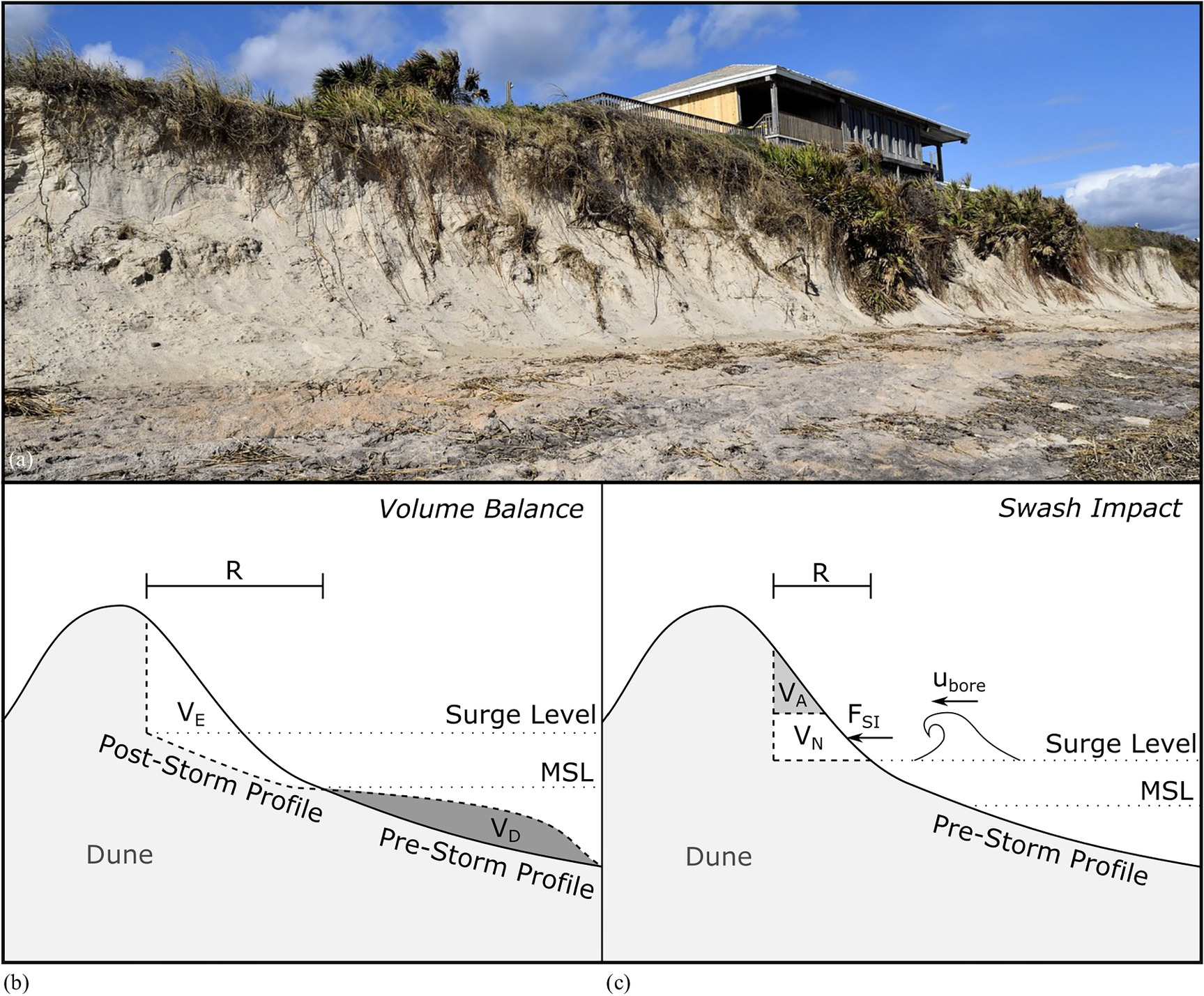
Fig. 4. Beach and dune erosion: (a) image of beach and dune erosion from Hurricane Matthew
[Image courtesy of Pixabay/Paul Brennan, under CC0 1.0 Universal Public Domain Dedication license (https://creativecommons.org/publicdomain/zero/1.0/)]; (b) volume balance approach that predicts dune recession (R) by equating the erosion volume (VE) and deposition volume (VD), (adapted from Edelman 1972); and (c) swash impact approach that relates wave bore velocity (ubore) to the swash impact force (FSI) which creates notching (VN) that leads to avalanching (VA), (adapted from Nishi and Kraus 1996).
Other analytical methods were developed to predict beach and dune erosion using similar assumptions; these models included DUROS (Vellinga 1986), as well as those of Kobayashi (1987), Dean (1991), and Kriebel and Dean (1993). Fundamentally, each of these models is similar in that they are based on balancing eroded and deposited sediment volumes, while the main differences lie in the factors that influence the new profile shape. For example, the profile depth in the nonlinear Provisional Method was considered only a function of distance from the shoreline (Edelman 1972), while other methods allowed the depth to adjust based on factors such as wave height and sediment characteristics (e.g., Vellinga 1986; Dean 1991). Komar et al. (1999) also developed a simple method to predict dune retreat based on the foreshore slope and the height of the runup above the dune toe; this approach has been recommended by FEMA for United States Pacific Coast beaches as of 2005 (Mull and Ruggiero 2014). Vellinga’s (1986) DUROS model continues to be used in the Netherlands to assess the health and safety of the coastal dunes (Bosboom and Stive 2021).
One important limitation with these early models arises from the assumption of instantaneous response. Because the duration of a storm is often much shorter than the time required for profiles to erode to their new equilibrium states, they rather erode some fractional amount toward equilibrium but never reach it. Komar and Moore (1983) put it succinctly, stating that these methods “should be regarded as an upper limit or an erosion potential that would result if the storm conditions were held constant indefinitely.” For conservative estimates and design standards, these methods may prove reliable. However, for higher levels of modeling accuracy, it may be necessary to shift toward time-dependent models or the combination of idealized models with a time-dependent function (e.g., Kriebel and Dean 1993).
Fisher and Overton (1984) proposed another type of modeling approach that focuses on the impact of swash on the dune face. These are appropriately called “Swash Impact” approaches. The main idea undergirding this approach is that erosion of the dune is proportional to the impact force of colliding waves, which can be related to the waves’ bore heights and approach velocities [Fig. 4(c)]. Through a series of laboratory experiments, linear relationships were found between the amount of dune erosion and swash impact force, modulated by statistically significant factors such as grain size and dune density (Overton et al. 1988, 1994). This relationship was also identified in the field through a series of experiments at Duck, North Carolina (Fisher et al. 1987).
Other methods using this approach were developed by Nishi and Kraus (1996), Larson et al. (2004a), and most recently by Palmsten and Holman (2012). Nishi and Kraus (1996) calculated the swash impact force by multiplying the mass of water in the approaching wave by its deceleration upon impact. Using large-scale wave tank experiments on compacted and uncompacted dunes, they found linear relationships between the weight of eroded sediment and the impact force. They also found uncompacted sediment to be more susceptible to swash impact erosion and suggested artificially compacted dunes as a possible method of erosion control. Using the linear relationship between erosion and swash impact force as an initial assumption, Larson et al. (2004a) derived an analytical model that predicted dune recession as a function of bore speed, initial geometry, empirical transport coefficients, and foreshore slope, which was assumed to linearly continue landward of the dune toe. The authors used four previously published datasets to test their model and to empirically derive an optimal transport coefficient. Lastly, Palmsten and Holman (2012) improved on this formulation in two main ways: (1) they used a Gaussian distribution to model variability in wave runup elevations; and (2) they tested various runup exceedance values and found that using a runup exceedance value of 16% led to better dune erosion predictions in the laboratory when compared to the 2% runup exceedance guidance recommended by Sallenger (2000).
Modeling Shoreface Response
Paralleling these advancements was the development of more complex sediment transport formulations. While these formulations may vary in approach, they are similar in that they relate hydrodynamic parameters (e.g., velocity) to sediment transport rates. Thus, for the purposes of this discussion, we refer to these more complex formulations as coupled hydrodynamics–sediment transport (HD–ST) formulations. Since a review of each formulation would take considerable space, we offer a cursory description the HD–ST formulations and refer interested readers to Larson and Kraus (1989), Dean and Dalrymple (2002), Nielsen (2009), Aagaard and Hughes (2013), Bosboom and Stive (2021), and references therein for additional details.
In highly resolved models, coupled HD–ST formulations use hydrodynamic parameters to predict both bed load and suspended load transport rates. Bed load transport is typically estimated as a function of the bed shear stress, sediment density, and average grain diameter (often using Shields parameter), whereas the suspended sediment transport rate is calculated by integrating the vertical velocity and concentration profiles, the latter of which can be based on functions such as the Rouse profile or advection–diffusion calculations (Bosboom and Stive 2021).
Depending on the application, not all models can afford the computational burden associated with coupled HD–ST formulations. Other approaches with less computational burden have gained popularity, such as the equilibrium-based approach, originally developed by Kriebel and Dean (1985), which assumes the existence of an equilibrium shoreface profile that controls how the shoreface responds under specific hydrodynamic conditions. It is founded on the idea that if hydrodynamic conditions remained constant, then the shoreface would respond until constructive (landward) and destructive (seaward) forces along the profile were balanced, leading to a steady profile with an XST rate of zero. Kriebel and Dean (1985) developed a formulation that calculates an equilibrium profile based on depth-dependent energy dissipation rates. XST rates are then calculated at a particular shoreface depth based on the difference between the actual energy dissipation rate and the rate associated with the equilibrium profile (Dean and Dalrymple 2002).
Another popular approach is the energetics approach, which was originally developed by Bagnold (1963) for fluvial sediment transport. This approach considers the hydrodynamic environment as a machine that performs a certain amount of work (sediment transport) based on the available power input (kinetic energy) modulated by some efficiency factor (resistance to transport) (Bagnold 1966). Bed load and suspended load transport rates are calculated separately based on the available wave power, or the wave energy flux per unit width, which drives the transport (Dean and Dalrymple 2002). While the energetics approach has been successful in predicting offshore-directed sediment transport rates during storm events, this approach has generally underpredicted onshore sediment transport during recovery periods (Aagaard and Hughes 2013).
Modeling Overwash
Efforts to quantitatively understand and predict overwash have led to the development of various formulations, which generally fall into one of two categories. Those in the first category may be described as “Bulk” approaches, as defined by Donnelly et al. (2006), since they relate certain hydrodynamic parameters (e.g., wave height) to bulk washover volumes [Fig. 5(a)]. Williams (1978) published the earliest bulk formulation, which predicted the washover rate as a function of excess runup (i.e., depth of runup over the dune crest) and wave period. Later bulk formulations (e.g., Tanaka et al. 2002) were based on laboratory experiments by Kobayashi et al. (1996), which showed a linear relationship between overwash and washover rates. Formulations in the second category apply coupled HD–ST formulations, which were discussed in the previous section. Donnelly et al. (2006) reviewed at least three of these formulations and their results, including Leatherman (1976) who coupled the Einstein transport equation to velocity measurements, Sánchez-Arcilla and Jiménez (1994) who combined the Van Rijn formulation with velocities calculated using the Chezy equation, and Baldock et al. (2005) who applied a standard sheet flow model based on Shield’s parameter to calculated swash velocities.

In the last fifteen years, most overwash modeling efforts have been directed toward developing, improving, and applying the coupled HD–ST formulations, which are typically just one component of event-scale morphodynamic models that resolve multiple sediment transport processes at small spatial scales. At the time of Donnelly’s (2006) review, only one such model (i.e., SBEACH) was able to predict overwash. The original formulation, developed by Wise et al. (1996), predicted sediment transport landward of the swash zone boundary based on the estimated wave bore velocity at the dune crest, and interpolated the transport rate to both landward and seaward limits. This formulation was later updated by Larson et al. (2004b) who modified landward flow dissipation by including a lateral spreading component, and Donnelly et al. (2005, 2009) who used the Sallenger (2000) regimes to model intermittent overwash by wave runup and quasi-steady overwash during barrier inundation, the latter of which used a standard weir equation. Donnelly et al. (2005, 2009) compared the updated model results to posthurricane field data at Assateague Island, Maryland, Folly Beach, South Carolina, and Garden City Beach, South Carolina, showing good agreement with the poststorm profiles. In addition, Donnelly’s model was shown to outperform that of Larson et al. (2004b) in predicting the poststorm profile at Assateague.
Recent work has also involved the incorporation of bulk overwash formulations into long-term and large-scale barrier evolution models. The long-term model of Jiménez and Sánchez-Arcilla (2004) employs a bulk formulation for modeling overwash rates based on empirically derived annual overwash volumes. This formulation uses the critical length concept of Leatherman (1979), which posits a theoretical threshold (i.e., critical barrier width and height) at which overwash is prevented. Deviations from these critical thresholds are used to estimate accommodation space (or volume) in the subaerial and backbarrier zones [Fig. 5(b)]. Thus, event-driven overwash is modeled continuously and quantified by the available accommodation space up to some predetermined maximum annual overwash volume. More recent models (e.g., Lorenzo-Trueba and Ashton 2014; Lorenzo-Trueba and Mariotti 2017) also use the critical length concept to model overwash in their long-term models.
Larson et al. (2009) followed a different approach, developing an analytical method to simulate the retreat of the barrier (or dune) based on landward (i.e., overwash) and seaward (i.e., profile erosion) sediment fluxes. Using a triangular approximation for the island or dune, these flux values were correlated with the ratio of dune crest to total runup elevations, and validation with field data showed results could provide order-of-magnitude estimations of overwash flux.
Modeling Breaching
In modeling a breach, there are a number of important components that one may wish to consider including the location of breach occurrence, the timing of breach formation, breach dimensions and their progression (i.e., expansion or contraction), and finally its ultimate state (e.g., natural closure, stable inlet). While there has been some quantitative work on predicting systematic breach occurrence (e.g., Kraus et al. 2008) and long-term inlet stability (e.g.,Kraus and Wamsley 2003 and references therein), our focus will be limited to models with strong morphodynamic components (i.e., breach formation, initial breach growth, and long-term progression).
Visser (1998) developed a conceptual model of breach formation and initial growth. Although the model was originally developed for sand dikes, it can also be applied to barrier islands. The conceptual model described five phases: (1) erosion and steepening of the inner slope of the scour channel; (2) decreasing of the crest width; (3) crest lowering and breach widening; (4) breach widening as flow changes from critical flow to subcritical flow; and (5) breach widening during subcritical flow until the flow ceases. This conceptual model was translated into BRES, a numerical model that predicts breach formation and initial growth based on discharge (calculated using the broad-crested weir equation) through an initial trapezoidal cross-section (Visser 2000). Testing against multiple laboratory and field studies, Visser (2000) found good agreement between predicted breach widths over time and measured data.
Basco and Shin (1999) published a 1D numerical breaching model based on storm stages, in a similar fashion to Sallenger’s (2000) regimes. Dune erosion was modeled in the first stage, followed by a diffusion-based approach to overwash in the second stage. The third stage aligns with Sallenger’s inundation regime, while the fourth stage aligns with the outwash regime. In these last two regimes, barrier inundation and breaching were modeled by combining the 1D Saint-Venant equations with the sediment transport formulation of Van Rijn (1984). This approach to breach modeling has been included in more recent event-scale morphodynamic models (e.g., Delft3D, XBeach), which combine hydrodynamic output with specific sediment transport formulations. These models predict breach formation during barrier inundation, when flow velocities across the island scour antecedent low spots into fully formed channels. Additional details on these models may be found in the following section.
Kraus (2003) developed an analytical breaching model that predicts the development of a rectangular breach toward equilibrium dimensions using an exponential time function. The model starts with some initial channel or nonuniformity in the dune or island and proceeds toward a full breach based on flow through the channel which erodes the channel bed and sides. Kraus (2003) found the breach response to be sensitive to initial channel dimensions. Kraus and Hayashi (2005) later expanded the model to include a coupled HD–ST formulation, where breach progression was based on calculated bottom and critical shear stresses. The model was shown to reproduce general trends of an observed breach, yet it tended to underestimate the breach width and overestimate the breach depth (Kraus and Hayashi 2005).
A more recent analytical breaching model was developed by Nienhuis et al. (2021) that is based on the hypothesis that a breach develops when the volume of sediment transport by overwash exceeds the sediment volume stored in the subaerial island. Overwash volume is calculated analytically using a triangular storm surge time series and integrating an overwash flux equation that considers surge height, width, and depth of the dune gap, and a friction coefficient to account for vegetation impacts. Nienhuis et al. (2021) compared their model results to Delft3D simulations and found that it performed reasonably well, although the Delft3D simulation predictions varied across one additional order of magnitude compared to the analytical model. Results were also compared with observations from Hurricane Sandy which showed that the model performed much better for undeveloped barriers as compared to developed barriers.
Multifaceted Event-Scale Modeling
A variety of morphodynamic models have been developed to simulate more than one event-scale phenomena/process—we refer to these as “multifaceted” models. Readers familiar with the literature will recognize that many of these multifaceted models are commonly called “process-based” models, although we have intentionally avoided this term due to its inconsistent and ambiguous usage in the literature, as well as its implication that more abstracted models are not based on processes. We now present select event-scale models, followed by a brief discussion of multifaceted modeling efforts related to storm sequencing and poststorm recovery, which has received less attention from researchers until recently.
Event-Scale Models
While a variety of multifaceted event-scale models exist, herein, we focus on models that have been thoroughly cited in the literature and are widely used by the coastal morphodynamics research community. These include models such as SBEACH (Larson and Kraus 1989), which rely on equilibrium concepts, and models such as DUROSTA (also known as Unibest-DE) (Steetzel 1993), CShore (Kobayashi et al. 2008), Delft3D (Lesser et al. 2004), and XBeach (Roelvink et al. 2009), which are based on coupled HD–ST formulations. Some of the primary differences between these models are shown in Table 3, including model dimensionality, included processes, and process formulations. We discuss the development of each model and highlight some significant improvements. Readers are referred to the references provided with each model for additional details.
Process Formulationsa | ||||||
---|---|---|---|---|---|---|
Model Name (Reference) | Dimensions | XST | LST | OW | BR | Model Description |
SBEACH (Larson and Kraus 1989) | 1D | KD85 | WIS96 | XST rates estimated through semiempirical relationships in shoreface regions; considers wave and sediment characteristics, wave shoaling, breaking, setup and setdown, breaker decay and reformation, sediment slumping/avalanching. | ||
DUROSTA/Unibest-DE (Steetzel 1993) | 1D/Q2D | […STZL93…] | Only considers suspended load transport (bed load neglected); considers wave set-up, energy dissipation from bed friction after breaking with a turbulence model; employs a bed slope correction factor and extrapolates swash transport rates based on calculated rates at the wet/dry interface. | |||
CShore/C2Shore (Kobayashi and Farhadzadeh 2008; Grzegorzewski et al. 2013) | 1D/2D | [….KBY08….] | KBY10 | Hydrodynamic components include the combined action of incident waves and currents, considering wave shoaling, breaking, and roller energy; considers shoreface (or structure) permeability and overtopping using an empirically based, probabilistic runup model. | ||
Delft3D (Lesser et al. 2004) | 2D/3D | [………………VRN93*…………….] | Shallow water equations solved in 2D (depth-averaged) or 3D; allows coupling to HISWA or SWAN wave models which consider breaking, bed friction, and streaming (near-bed currents); includes surface roller and infragravity formulations; includes bed slope correction and morphological acceleration factor. | |||
XBeach (Roelvink et al. 2009) | 2D | [………………SVR97*…………….] | Depth-averaged shallow water equations solved in Sallenger’s (2000) storm impact regimes; includes wave breaking, swash dynamics (modeling wave groups, infragravity waves, surface rollers, and return flows), beach and dune erosion (including avalanching), overwash (using low-frequency wave group forcing), and breaching by channel scouring. |
SBEACH (Larson and Kraus 1989) was developed in the late 1980s to predict profile response to storm events. The model employed the XST formula of Kriebel and Dean (1985), which is based on the difference in energy dissipation between the actual profile and an equilibrium profile. The model was originally calibrated using data from large wave tank experiments, showing its ability to predict foreshore erosion and bar formation, and its inability to predict features landward of the bar such as the trough and berm development during accretionary simulations (Larson and Kraus 1989). The original model (which did not include overwash) was formally updated with the overwash formulations of Wise et al. (1996) and again by Larson et al. (2004b), who showed good agreement between model predictions and measured profile changes for observations at Ocean City and Assateague, Maryland. SBEACH has more recently been incorporated in economic models for evaluating beach nourishment projects (e.g., Gravens et al. 2007), probabilistic frameworks for predicting erosion (e.g., Callaghan et al. 2013), and model comparison studies, where it produced better morphological predictions than XBeach when using default parameters, but underperformed when calibration data were employed (e.g., Callaghan et al. 2013; Simmons et al. 2019).
DUROSTA, which is an acronym in Dutch for “dune erosion—time dependent,” was developed in the early 1990s as an unsteady, numerical model upgrade to the analytical beach and dune erosion models DUROS (Vellinga 1986) and DUROS+ (the “+” representing the addition of wave period to the original model parameterization). The model was initially validated by comparison to laboratory data and various field experiments and showed good prediction capabilities on the subaqueous profile while underestimating dune retreat (Steetzel 1993). DUROSTA was used by Van Baaren (2007), who found that wave period, bed slope, and the location of transition between the wet and dry profile zones were important model parameters. Hoonhout (2009) also used the DUROSTA model to study the effects of shoreline curvature on dune erosion and retreat during storm events, finding that consideration of shoreline curvature significantly impacted the model results. Currently, DUROSTA and another cross-shore model Unibest-TC (Ruessink et al. 2007) are optional modules that may be employed when using the one-line model Unibest-CL+.
De Goede (2020) presented a historical review of the development of Delft3D, from initial 2D shallow water code development in the late 1960s, to coupling of updated wave models (e.g., SWAN), to the addition of turbulence closure models for 3D flows in the 1990s, and finally the incorporation of sediment transport formulations into the hydrodynamic module. Lesser et al. (2004) presented details on the latter update, as well as the inclusion of a morphological acceleration factor for long-term simulations and validation studies showing that the results compared well to analytical solutions, laboratory data, and other accepted numerical model solutions. Delft3D is widely used in both practice and research (De Goede 2020), including studies on event-scale flooding (e.g., Cañizares and Irish 2008), storm sequence morphodynamics (e.g., Alfageme and Cañizares 2005), breach stability and growth (e.g., Alfageme et al. 2007), and morphodynamic changes between storm events (e.g., van Ormondt et al. 2020).
Johnson et al. (2012) presented a thorough summary of the historical development of CShore from its initial goals in modeling nonlinear wave transformation in the late 1990s, to aiding in coastal structure design, and finally its development toward modeling nearshore morphodynamics in the late 2000s. Johnson et al. (2012) also provided results from sensitivity analyses, model calibration, and validation at nine field sites, which showed the model was capable of producing reasonable estimates of event-driven morphological changes, while tending to underpredict dune erosion and retreat. Work and improvement on the model has continued through at least 2015 (Kobayashi 2016), and the model has also been extended to two dimensions (C2Shore), the latter of which was validated through simulations of morphological response to Hurricane Katrina at Ship Island, Louisiana (Grzegorzewski et al. 2013). CShore does not explicitly model sheet flow or ebb currents, reducing its applicability during barrier inundation (Harter and Figlus 2017).
XBeach is considered the state-of-the-art event-scale model to predict barrier response to storm events. Lead by Roelvink et al. (2009), XBeach was developed as an open source model to predict all of the main morphological responses associated with storm events (i.e., beach and dune erosion, overwash, and breaching) corresponding to the storm impact regimes of Sallenger (2000). Model validation studies showed it was able to predict storm hydrodynamics and morphological responses well (Roelvink et al. 2009), although subsequent studies have shown that high simulated velocities in the swash zone consistently led to slight overpredictions of erosion near the dune toe (e.g., van Dongeren et al. 2009; De Vet 2014). To correct these overpredictions, researchers have attempted to artificially lower sediment mobilization (by modifying the critical Shield’s number); however, while this led to more accurate predictions of dune toe erosion, it decreased the accuracy of breaching simulations (De Vet 2014). Elsayed and Oumeraci (2017) found that modifying suspended sediment concentrations based on the local bed slope helped to resolve this issue. Some of the most recent work with XBeach has involved modifying roughness coefficients. Passeri et al. (2018) implemented spatially varied roughness coefficients based on land cover, which showed improved morphodynamic predictions over simulations with constant roughness values. Alternatively, van der Lugt et al. (2019) implemented dynamic roughness values that vary during the simulation according to erosion and deposition patterns, which showed improved results over simulations with static roughness values.
Many of these event-scale models continue to be tested and applied today. Although XBeach has become the standard for modeling event-scale morphodynamics, recent comparison studies indicate that other models (e.g., CSHORE, SBEACH, Delft3D) are also being used and evaluated for their strengths (e.g., Harter and Figlus 2017; Simmons et al. 2019; Cho et al. 2019). Furthermore, various studies have loosely coupled these event-scale models together to utilize the strengths of each model. For example, Cañizares and Irish (2008) used SBEACH to simulate dune erosion and lowering prior to inundation and breaching using Delft3D. XBeach and Delft3D have also been loosely coupled in a recent breaching study by van Ormondt et al. (2020), who used XBeach to simulate breaching development during the storm and Delft3D to simulate breach development and growth after the storm event.
Model coupling has also been used in the development of new modeling systems. The COAWST modeling system, which was developed by coupling a regional ocean model (i.e., ROMS), a nearshore wave model (i.e., SWAN), and an open source sediment transport model (i.e., CSTMS) (Warner et al. 2010), is appearing more frequently in the coastal morphodynamics literature, including specific application to shoreline change modeling (e.g., Safak et al. 2017) and barrier islands (e.g., Safak et al. 2016; Warner et al. 2018). Numerous other modeling systems have been developed (e.g., Kaveh et al. 2019), but have yet to gain a literature foothold in this particular field of study.
Storm Sequences and Poststorm Recovery
Some of these event-scale models have also been applied to the study of storm sequences, which investigates the nonlinear impact of sequential storms on beach and dune erosion, where successive smaller storms have a cumulative effect that exceeds the impact of an independent event (Senechal et al. 2017). Various modeling studies have been conducted to quantify this cumulative impact and to determine the most important driving factors such as antecedent beach states (e.g., Splinter et al. 2014) and the order of the most severe storms within the sequence (e.g., Dissanayake et al. 2015).
Based on a survey of the literature, Eichentopf et al. (2019) identified three primary conceptual descriptions to aid in modeling the impact of storm sequences, and discussed evidence from published studies for each description. The three conceptual descriptions are: (1) initial storm destabilization, where the first storm in the sequence erodes the beach, leaving it more vulnerable to the next storm event; (2) extreme storm impact, where the largest storm event of the sequence is of primary importance regardless of storm order; and (3) benchmark storm impact, where all events in a storm sequence may be combined and modeled as a single large storm event, similar to a benchmark or design storm approach in hydrologic analysis. Various types of models that have been employed and/or developed to study storm sequences including statistical models (e.g., Pender and Karunarathna 2013), long-term equilibrium-based models such as ShoreFor (Davidson et al. 2017) or PCR (Ranasinghe et al. 2012a), and multifaceted event-scale models such as XBeach and Delft3D (e.g., Splinter et al. 2014; Dissanayake et al. 2015).
In addition to reviewing the literature on storm sequencing, Eichentopf et al. (2019) also provide a brief section on recovery, which they indicate is much less studied than the impact of storm sequences. They concluded with recommendations for future research, which broadly included additional physical and numerical simulations, improved data collection efforts, and stronger research emphasis on beach recovery processes.
Summary of Advancements and Limitations
The practice of modeling event-scale barrier morphodynamics has followed a natural progression from conceptualizing models based on observations, to the creation of simplified and efficient rule-based models, to the development of more complex sediment transport formulations coupled with hydrodynamic calculations at fine spatiotemporal scales. Reconsidering our Grand Challenge statement, it is apparent that significant advancements have been made over the last fifty years. The earliest and most basic models (e.g., analytical dune erosion models) were intuitive, easy to use, and could provide conservative estimates for dune recession and likelihood of failure. Empirical studies followed, which advanced our ability to quantify the impact of key processes based on hydrodynamic output (e.g., predicting notching/avalanching of the dune face based on swash impact, predicting overwash volumes based on runup exceedance, predicting sediment transport rates based on velocity and concentration profiles, etc.). This improvement in scientific understanding, along with the advancements in computing power, has allowed us to continue reducing the spatiotemporal scales of our morphological predictions while maintaining or increasing accuracy.
However, there are still major limitations to our modeling capabilities. Although the accuracy of simulations has improved, we are still a long way from high confidence predictions. This is partially due to the scarcity of data to evaluate the predictive capability of models mid-storm. Event-scale models are able to capture the general trends of erosion and deposition compared to prestorm and poststorm profile (or LiDAR) data; however, the small-scale predictive abilities of our models during storm is largely unknown since there is little to no data to validate those predictions. Our apparent distance from high-confidence predictions can also be attributed to both epistemic uncertainty (i.e., that which arises from our lack of knowledge of the relevant processes) and intrinsic uncertainty (i.e., that which arises from the inherent randomness of natural processes). For example, we know that some factors—such as vegetation and anthropogenic impacts—play an important role in event-scale morphodynamics, yet the modeling of such factors is (for various reasons) still in its infancy. In addition, the inherent randomness of forcing conditions (e.g., storm characteristics, wave climates) and initial conditions (e.g., bathymetry, sediment characteristics) is difficult to capture at smaller scales.
Long-Term Morphodynamics
This section provides an overview of commonly modeled phenomena and processes associated with long-term morphodynamics, a review of relevant modeling efforts, and a summary of advancements toward the Grand Challenge.
Commonly Modeled Phenomena and Processes
During the periods of time in between storm events, chronic sediment transport processes resume their work that contributes to gradual morphological change. The following sections discuss commonly modeled long-term phenomena (i.e., shoreline change and barrier transgression) and relevant morphodynamic processes.
Shoreline Change
The shoreline can be smoothed or caused to vary in form depending on the angle of the incident waves which drive LST (Ashton et al. 2001). Thus, shoreline change is observed as the local shoreline is moved either landward or seaward by gradients in LST rates. These gradient-driven changes can also manifest themselves in other ways including island migration, barrier elongation, inlet migration, and island dimensional changes.
Although it is not as common, entire barrier islands can migrate in the direction of LST when sediment is eroded from the updrift end, carried alongshore, and deposited at the downdrift end, assuming no updrift sediment sources. Otvos (1970) noted this phenomenon in the northern Gulf of Mexico by observing that barriers can migrate large distances (i.e., several kilometers) from their location of origin. When the barriers are stable and not prone to migration, newly formed inlets may migrate instead. This phenomenon results from an LST gradient across the inlet, where sediment is deposited updrift of the inlet and eroded downdrift.
Dimensional changes may also be observed due to LST gradients and the placement of engineering structures. McCann (1979) observed that most islands developed greater widths on the downdrift end of the island as compared to the updrift end, which was attributed to a minimal amount of updrift sediment available for transport. If a continuous source of updrift sediment is present, and sediment is not removed from the barrier system, then barrier elongation could be observed as sediment is continually added to the downdrift end. Penland and Boyd (1981) described lateral migration of barrier islands and the influence of placing coastal structures at various locations along the islands. For example, structures placed near the updrift end tended to reduce the total island area while structures placed in the middle of the island tended to increase the total area.
Barrier Transgression
In addition to shoreline change, most barrier islands are undergoing transgression (i.e., landward migration) in accordance with SLR. However, this migration did not appear to be widely accepted in some of the earliest literature (e.g., Schwartz 1973; Leatherman 1987). Nevertheless, once transgression was recognized by the research community, many studies sought to identify the driving mechanisms that were primarily responsible for it. Otvos (1970) indicated that overwash and aeolian processes were primarily responsible for the landward movement, which was supported by others such as Moody (1964), Godfrey (1970), and Leatherman (1987). Others found sediment transport through tidal inlets and/or breaches to play a much larger role (e.g., Pierce 1969; Armon and McCann 1979; Fisher and Simpson 1979; Leatherman 1979; USACE 1984).
SLR rate is also considered one of the primary drivers of barrier transgression through its interaction with storm processes such as overwash and breaching. Although not developed specifically for barriers, the Bruun Rule (Bruun 1962) exemplifies the theorized direct relationship between SLR and shoreline transgression. The interaction between rates of SLR and other transgressive processes was published in an interesting study by Moslow and Heron (1979). They found that previous high rates of SLR were correlated with dominating overwash processes and high rates of transgression. Conversely, when the rate of SLR slowed, they found that transgression also slowed and inlet dynamics became the dominant method of sediment transport between the ocean and backbarrier environment.
During landward transgression, barrier islands may also maintain their elevation with respect to SLR through the combination of overwash and inlet dynamics/breaching. As SLR effectively reduces barrier island relief, barriers are more prone to overwash and inundation during storm events, which deposit sediment on the island or behind it (i.e., washover deposits). This deposition effectively translates the island landward and increases its elevation. As this process is sustained, the barrier sediment may be conceptualized as “rolling” over itself, which has led to the description of this cycle as “barrier rollover” (Moore and Murray 2018). Lorenzo-Trueba and Ashton (2014) referred to this sustainable behavior as dynamic equilibrium.
Similarly, lagoonal washover deposits and flood tidal shoals have been shown to assist the barrier in maintaining its elevation through the reduction of accommodation space for future washover (Stolper et al. 2005). For example, consider a salt marsh that grows on top of washover deposited in a lagoon during some initial storm event. When a subsequent storm arrives, sediment that would have been deposited in the lagoon is now deposited on top of the new salt marsh. Thus, the salt marsh (and previous washover deposit) acts to reduce the available lagoon space for washover, and elevation is increased in that location as a result. Recent modeling work has suggested that the presence of backbarrier marsh not only increases island elevations, but actually reduces landward transport by encouraging the subaerial deposition of sediments (Johnson et al. 2021). As the barrier continues its rollover toward the mainland, those previously buried marsh and lagoonal sediments may show up as shoreface outcrops which can affect the future morphodynamics through changes in the sediment supply (i.e., the source of sediment that feeds the growing barrier).
Although sustained barrier transgression is associated with increases in subaerial elevations with SLR, barriers may also lose elevation due to compaction of the underlying sediment. Hoyt (1969) was possibly the first to mention the idea of vertical movement by compaction or isostatic adjustment. He stated that “compaction or isostatic movement caused by weight of the sediment deposited in the coastal area may result in formation of lakes or lagoons by depression of the chenier plain below water level.” As the barrier rolls over previous marsh sediment, the marsh sediment compacts under the load of the island, inducing an even higher local rate of SLR.
Barrier island transgression is also considered to be influenced by two other factors: (1) the slope of the shelf over which it is migrating; and (2) the sediment supply. If we only consider the geometry of the system and assume that barriers maintain their dimensions, it is apparent that barriers must migrate at higher rates over shallower slopes to keep pace with SLR (Pilkey and Davis 1987). Numerous studies have concluded that antecedent topography is extremely important to the development and configuration of modern day barrier islands (e.g., Halsey 1979; Oertel 1979; Belknap and Kraft 1985). Others have concluded that sediment supply is more important to the rate of migration, with less sediment supply leading to increased migration (e.g., Swift 1975; Storms et al. 2002; Moore et al. 2007; Ruggiero et al. 2010). Dillon (1970) commented on the cross-shore migration of barriers through stratigraphy observations and concluded that barriers were not forced to continue landward migration with SLR, but could drown if the sea level advanced too quickly or if there was an insufficient supply of sand.
Modeling Efforts
Perhaps the most challenging question related to barrier morphology is “What will be the state of a barrier system 10, 100, or even 1,000 years from now?” Compared to analyzing and predicting short-term responses, there is considerably less evidence available (that is, evidence or data collected using our current era’s level of scientific certainty) to evaluate historical trends and make long-term projections. Stratigraphic observation and analysis may provide a partial glimpse of historical system states; however, it also requires assumptions and a hermeneutic to make the evidence meaningful, thereby reducing the certainty of conclusions that may be drawn. However, there are also problems when extrapolating small-scale processes to large spatiotemporal scales (i.e., the problem of error propagation). Thus, the problem of long-term morphological analysis and prediction is not a trivial one, especially since it is closely tied to uncertainties surrounding climate change (e.g., future SLR and changes in storminess). Numerous publications from the early 1990s into the early 2000s discuss the philosophy behind long-term morphological prediction. The interested reader is referred to Stive et al. (1990), Terwindt and Battjes (1990), De Vriend (1991b), Latteux (1995), and Cowell et al. (2003b) for further details on this topic.
Similar to the previous section, the review of long-term morphodynamic modeling efforts is broken down according to the primary intent of each model. Thus, modeling efforts are categorized by those which model: (1) shoreline change; (2) shoreface evolution; (3) barrier transgression; and (4) phenomena that are typically combinations of categories (1)–(3). To assist the reader in keeping track of the models discussed, Fig. 6 offers a graphical representation of long-term models, in the chronology of their publication, that simulate some combination of shoreface evolution, shoreline change, dune growth/erosion, or overwash. Table 4 is a comprehensive summary of the long-term models discussed in this review, which includes each model’s relevant processes.
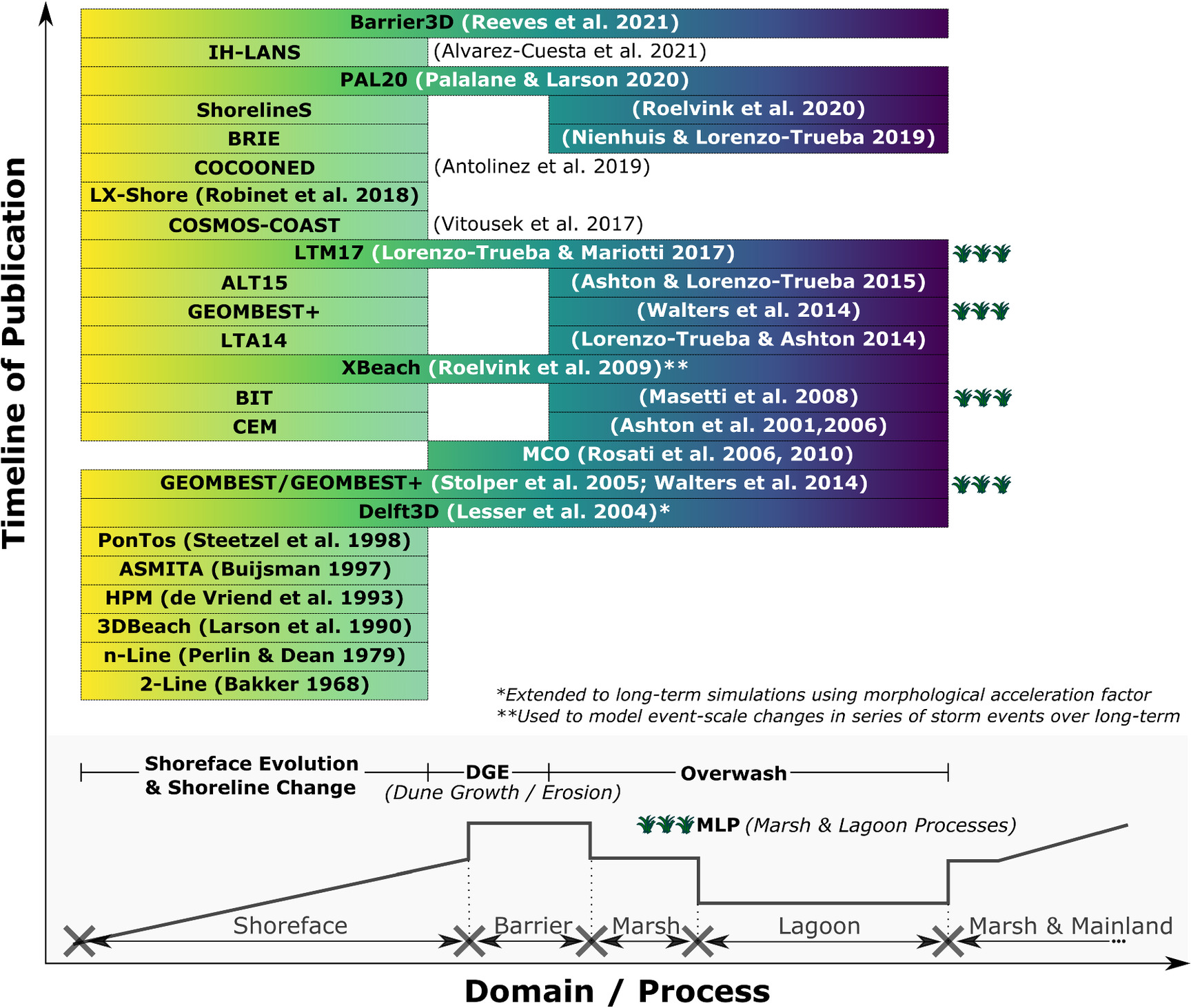
a
SFC, shoreface change; LSC, longshore shoreline change; TRN, transgression; ID, inlet dynamics; DGE, dune growth or erosion; SUB, subsidence; OW, overwash; MLP, marsh and lagoon processes.
Modeling Shoreline Change
Long-term modeling of shoreline change is often referred to as “shoreline evolution” modeling since the most observable impact of LST gradients is shoreline displacement, either landward or seaward. The first approach to modeling shoreline evolution stemmed from the one-line theory, published by Pelnard-Considere (1956). Models derived from this theory, commonly called “one-line models,” assume a constant equilibrium profile and calculate position changes in a single contour line—the shoreline—over time considering only the gradients in the LST rate [Fig. 7(a)].

Fig. 7. One-line and two-line model schematics: (a) one-line approach that predicts shoreline changes based on LST gradients (qx(j+1) − qx(j)); and (b) two-line approach that predicts change at the shoreline and an offshore contour, considering LST gradients in each zone and rule-based XST.
(Adapted from Perlin and Dean 1979.)
Larson et al. (1987) published a review of one-line modeling theory and analytical solutions that had been developed for various coast-specific and structure-specific situations. Two years later, Hanson and Kraus (1989) presented the one-line model GENESIS, which would become one of the most widely used one-line models for predicting shoreline evolution in practice, though not without criticism (Young et al. 1995; Houston 1996). One-line models are still being developed and used today, likely due to their simplicity, intuitiveness, and ease of calculation. The coastal evolution model (CEM) of Ashton et al. (2001) is a one-line model that predicts shoreline response due to high-angle waves, assuming a constant linear shoreface out to an estimated closure depth. From numerical experiments, they found that high-angle waves cause small shoreline perturbations to grow into larger formations, such as cuspates and spits. In addition, they found that shoreline protrusions can shelter downdrift features from the high-angle waves, affecting the evolution of such features. Thomas and Frey (2013) and Kim et al. (2020) reviewed other common one-line models including UNIBEST-CL+ (Deltares 2021), GenCade (Frey et al. 2012), which is a combination of GENESIS and the regional Cascade model (Larson et al. 2002), and the proprietary LITPACK model. These models include advances such as coupling XST formulations, wave transformation, and wave–current interaction. Notably, GenCade includes advances to model tidal inlet evolution and inlet dynamics such as inlet bypassing and inlet feature (e.g., shoal) sediment balance.
Bakker (1968) was unsatisfied with the one-line theory’s assumption of parallel bathymetric contour lines near engineered structures due to the apparent discontinuity it produced. In 1968, Bakker published a two-line model whereby XST could be approximated between two profile zones based on the profile’s deviation from an equilibrium state [Fig. 7(b)]. Perlin and Dean (1979) were the first to suggest expanding Bakker’s two-line approach to multiple lines, and followed up with publication of their n-line model six years later, which was named for its ability to handle a user-defined “n” number of contour lines (Perlin and Dean 1985). Although limited in their ability to produce nonmonotonically decreasing profiles, these models were the first to add elements of cross-shore change to one-line models, paving the way for later n-line models that would attempt to integrate both XST and LST (e.g., Steetzel et al. 1998).
Buijsman (1997) published the ASMITA model, which simulated interaction between the adjacent shoreline and tidal inlets. The model consisted of five nodes that represented the tidal channel, ebb shoal, flood shoal, and the adjacent shorelines. Sediment flux between these nodes was calculated based on equilibrium formulations of each feature. A similar approach was incorporated into the regional barrier island model called Cascade, presented by Larson et al. (2002). While ASMITA focused on modeling the channel evolution, Cascade focused on modeling the regional shoreline position over long time scales, but accounted for the dynamic inlet features in the form of sediment source and sink terms. Larson et al. (2002) applied Cascade to a regional stretch of a U.S. East Coast barrier island and found the model was able to satisfactorily predict the shoreline position updrift and downdrift of two inlets.
Modeling Shoreface Evolution
Although long-term modeling of barrier transgression was well underway by the 1980s, most models assumed a constant profile shape. It was not until the mid-1990s that shoreface evolution began to be modeled, with the publication of the hinged panel model (HPM) (de Vriend et al. 1993) and the advection–diffusion model (ADM) (Niedoroda et al. 1995).
A conceptualized model of the shoreface profile by de Vriend et al. (1993) discretized the shoreface into three sections: (1) the upper shoreface; (2) the lower shoreface; and (3) the middle shoreface, which acted as a transition zone between the upper and lower zones. On the lower shoreface, profile movement was assumed to be negligible compared to the scales of interest, while the upper shoreface was assumed to be highly active out to the depth of closure (i.e., the transition point to the middle shoreface). The sections were considered to be rigid panels, which rotated about hinge points at the panel intersections based on the net sediment transport into or out of the panel zone. This led Cowell et al. (2003b) to refer to this model as the HPM. Stive et al. (1995) published a full treatment on HPM, which used Bowen’s energetics formulation for XST between the shoreface sections. They found that HPM produced reasonable hindcast simulations, and that the effect of substrate slope on profile evolution was only relevant at geologic time scales.
Niedoroda et al. (1995) published a similar model, the main difference being the continuous formulation of XST as compared to the paneled formulation of Stive and de Vriend (1995). The continuous formulation is depth-dependent and breaks down the transport into a bed load (i.e., advective) term and a suspended load (i.e., diffusive) term; thus, it was called the ADM model by Cowell et al. (2003b). Although Stive et al. (1995) and Niedoroda et al. (1995) do not apply their models to barrier coasts specifically, their work signifies advancement in cross-shore shoreface modeling and the increased importance of including cross-shore processes in long-term models.
Another class of models that simulate shoreface evolution is the equilibrium shoreline models, which have become increasingly popular for simulating event-based to interannual change. These models combine equilibrium-based formulations of shoreface evolution with shoreline change models (typically one-line models). The two most popular models include Yates et al. (2009) and the shoreline forecast (ShoreFor) model of Davidson et al. (2013). Both models demonstrate that beaches often respond directly to wave forcing (e.g., as quantified by wave energy or dimensionless fall velocity); however, the equilibrium response time scale (which is often longer than a single storm event) plays an exceedingly important role in the morphological evolution. Further, the extensive observations and developed model of Yates et al. (2009) show that beaches become increasingly resistant to erosion while in an eroded state.
Modeling Barrier Transgression
Models of shoreline change and shoreface evolution often produce a landward or seaward shift in the shoreline and/or profile based on gradients in the sediment transport rates. However, these models are not able to account for barrier transgression as an observed phenomenon. Thus, numerous models were developed to simulate long-term transgression based on cross-shore processes (e.g., overwash, breaching, inlet dynamics) and long-term forcing conditions (e.g., SLR).
Translation Models
Bruun (1962) introduced what is perhaps the most popular hypothesis about cross-shore transgression, which states that an equilibrium beach profile translates upward and landward with SLR while conserving sediment volume. Years later this became known as the “Bruun Rule” (Schwartz 1967). Because the profile is “translated,” these types of models are often called “translation models” in the literature, and many them have been developed since publication of the Bruun Rule.
The Bruun Rule (1962) predicts profile recession distance based on the amount of SLR and the average beach slope while conserving sediment. In subsequent examination of his theory, Bruun (1983) revisited the assumptions behind the model development and cautioned modelers who might attempt to apply the Bruun Rule in coupled alongshore models and progradational scenarios. Upon further review of the initial publications by Bruun (1962) and Schwartz (1967), several researchers have offered criticism of the way that the Bruun Rule (and the underlying equilibrium profile concept) is used in current models (e.g., Pilkey et al. 1993; Thieler et al. 2000). Conceding that some of the criticisms of Pilkey et al. (1993) were valid, Dubois (1993) stated that such models can still be useful in formulating research questions and site-specific equilibrium-based models. A more recent study by Wolinsky and Murray (2009) highlighted additional limitations of the Bruun Rule as applied to long-term simulations on the order of millennia.
Rosati et al. (2013) offered a review of field studies that attempted to validate the Bruun Rule (or modified forms of it). More recently, the Bruun Rule has been used to model both barred and bermed beach profiles in a laboratory setting (e.g., Atkinson et al. 2018). D’anna et al. (2021) recently presented a reinterpretation of the Bruun Rule that explicitly partitions shoreline recession into passive flooding of the beach profile and wave-driven reshaping components. Similarly, Troy et al. (2021) assessed long-term profile submergence versus Bruunian recession of beaches on the Great Lakes, a model environment to observe the effects of significant water-level variability, which serves as a proxy for future SLR.
The Bruun Rule has also been expanded since its initial publication. Dean and Maurmeyer (1983) presented the Generalized Bruun Rule, which expanded the original model to include the recession of barrier coasts specifically, and noted that greater recession rates were predicted due to the additional sand volume being deposited on the subaerial island and in the lagoon. The Bruun Rule was also expanded to include source and sink terms in the models of Everts (1985, 1987). Everts proposed that historical rates of SLR and shoreface retreat are preserved in the slope of the seaward profile, assuming that the profile is not significantly reworked by LST or tectonic deformation processes. Everts compared present and past ratios of SLR to shoreface retreat for five U.S. East Coast barrier islands and found that some barriers are in a narrowing state. Everts proposed that these barriers would continue to narrow until a critical width is reached, at which point landward migration of the island would begin. This theory employed the previously mentioned critical length concept, which was first proposed by Leatherman (1983) and has since been used in other models (e.g., Lorenzo-Trueba and Ashton 2014). Further modifications of the Bruun Rule were published by Rosati et al. (2013), who included an additional term representing XST in the landward direction by overwash and/or aeolian processes, and Dean and Houston (2016), who added a LST term and sediment source/sink terms to Rosati’s 2013 formulation.
Cowell et al. (1992) developed the shoreface translation model (STM), which allowed modelers to keep track of changes in stratigraphy, and was later used in conjunction with field observations to perform hindcasting simulations (Cowell et al. 1995). The STM was later expanded using a probabilistic framework to produce distributions of results that could be statistically evaluated in risk management frameworks (Cowell et al. 2006).
Most recently, McCarroll et al. (2021) published the ShoreTrans model, which follows similar profile translation methodology with a couple of distinctions and additions. First, the model uses measured profiles instead of parametric representations. Second, in addition to the profile translation, ShoreTrans has been modified to incorporate dune erosion and accretion, sediment flux between the upper (active) and lower (inactive) shoreface, as well as source and sink terms that can modify the sediment supply.
Other Transgression Models
More recent transgression models cannot simply be described as “translation” models, since they also simulate profile changes. For example, Storms et al. (2002) published an evolution model called BARSIM, which was intended to preserve the simulation’s erosion and depositional time history for comparison to observed shoreface stratigraphy. They describe BARSIM as a “process-response” model in which erosional and depositional mechanisms were modeled separately. Storms et al. (2002) conducted multiple numerical experiments and found that their model successfully captured several general observations: (1) increased grain sizes led to steeper shoreface slopes; (2) higher sediment supply values decreased retrogradation and increased the likelihood of aggradation or progradation; (3) higher SLR rates increased the likelihood of barrier overstepping; and (4) lower substrate slopes allowed for greater landward rates of migration.
Stolper et al. (2005) published the GEOMBEST model, which allows for depth-dependent shoreface adjustment toward a theoretical equilibrium profile, thus allowing the shoreface to temporarily exist in disequilibrium. GEOMBEST is also able to simulate heterogeneous stratigraphic units that can differ in erodability. Using the conceptual model of Cowell et al. (2003a), GEOMBEST divides each simulated coastal tract into three cross-shore zones (i.e., shoreface, backbarrier, and estuary). Stolper et al. (2005) used this model to estimate possible stratigraphic histories in both steep and gentle sloping environments, showing that quantitative estimates may be useful where historical data may be lost or otherwise unavailable. They also showed that substrate slope plays an important role when nonerodable outcrops are present. Specifically, they found that steep slopes lead to narrowing of the estuary and barrier drowning unless there is an external increase in sediment supply.
Based on sensitivity analyses with GEOMBEST, Moore et al. (2007) found that increasing the SLR rate and decreasing sediment supply led to increased barrier migration. Moore et al. (2010) also studied the Holocene evolution of U.S. East Coast barrier islands and found that the most vulnerable islands were large with less erodable substrates and gentle slopes. Brenner et al. (2015) confirmed these findings and also found that positive and negative feedbacks occur based on the slope of the substrate and island trajectory, and the composition of the substrate and backbarrier deposits; the negative feedback adjusts island trajectory to the substrate slope while the positive feedback leads to barrier width adjustments.
In studying the effects of compaction on barrier island migration, Rosati et al. (2006) developed the migration, consolidation, and overwash (MCO) model to predict the response of barrier systems to a series of storm events. The MCO model used the convolution method of Kriebel and Dean (1993) to predict responses when there was no overwash, and the numerical method of Donnelly et al. (2005) to estimate overwash volumes when water levels exceeded the berm height. Rosati et al. (2006) found that when consolidation was considered, there were considerable increases in migration distance and reduction of dune elevations. They found that increases in surge heights and deep-water wave heights also led to significant increases in migration and reduction of dune elevations. Rosati et al. (2010) updated the 2006 model to include the overwash formulations by Donnelly et al. (2009), and found that barriers on top of compressible substrates migrated much faster than barriers on noncompressible substrates, assuming a sufficient sand supply. They also found lower dune elevations and island volume loss to be more prevalent when compressible substrates were present, the thickness of which was found to be nonlinearly related to consolidation rates.
Masetti et al. (2008) developed the barrier island translation (BIT) model with separate sediment transport formulations for shoreface evolution, inner shelf reworking, overwash, and backbarrier infilling. They found barrier migration to undergo significant increases and decreases in migration rate according to the substrate slope and sediment availability. In addition, they found that offshore subaqueous bodies of sediment were most likely due to barrier migration over a nonuniform surface, rather than drowning of previous barrier islands.
Lorenzo-Trueba and Ashton (2014) developed a barrier island evolution model (hereafter “LTA14” model) to evaluate long-term behavior of the system. The model tracked transect boundary changes in the cross-shore direction based on sediment flux calculations. They found that barriers evolved following one of four behaviors: height drowning, width drowning, constant transgression (or dynamic equilibrium), and periodic transgression. Most recently, Reeves et al. (2021) expanded the LTA14 model domain to consider dune and subaerial island processes in a model called Barrier3D. The Barrier3D model used the LTA14 equations to simulate shoreline and nearshore profile change, and included additional formulations for dune growth during nonstormy periods, dune reduction by overwash, alongshore dune elevation changes, and sediment transport by overwash and backbarrier overland flow. Barrier3D also used probability distributions to simulate synthetic storm events and barrier recovery between storms (Reeves et al. 2021).
Multifaceted Evolution Models
Whereas most of the previously discussed long-term models were developed to simulate one primary phenomenon (e.g., shoreline change, shoreface evolution, barrier transgression), other recent models have been developed with the intent to simulate multiple long-term phenomena. We discuss four categories of these multifaceted evolution models: (1) coupled barrier-backbarrier models; (2) models that combine shoreline change and transgression; (3) models that combine shoreline change and shoreface evolution (i.e., equilibrium shoreline models); and (4) extended event-scale models.
Coupled Barrier–Backbarrier Models
In the last decade, barrier island evolution models have been coupled with backbarrier models to evaluate interactions or feedbacks between the systems. Walters et al. (2014) published GEOMBEST+, which coupled GEOMBEST with a backbarrier model from Mariotti and Fagherazzi (2010). Using this model, they found that overwash played an important role in that it provided a narrow platform for backbarrier marsh growth, which in turn reduced island migration rates by decreasing accommodation space for sediment deposition. Lorenzo-Trueba and Mariotti (2017) also developed a coupled model that combined the backbarrier marsh model of Mariotti and Carr (2014) and Lorenzo-Trueba and Ashton (2014). They found that including processes such as import/export of fine sediment to the barrier environment significantly impacted the accommodation space for overwashed sediment, which ultimately led to either a sustained island that migrated or one that drowned.
Models that Couple Shoreline Change and Transgression
Noting that most of the previous modeling efforts focused on either shoreline change or transgression, models are increasingly being developed to include both components. In 2006, the CEM model was updated to include a function for barrier overwash (Ashton and Murray 2006) and was later coupled with the LTA14 cross-shore barrier model (Ashton and Lorenzo-Trueba 2015). The authors found that when alongshore coupling was less significant, large alongshore variations persisted longer in the simulation; thus, alongshore coupling was found to act as a dampener on barrier transgression (Ashton and Lorenzo-Trueba 2015).
Nienhuis and Lorenzo-Trueba (2019) published the BarrieR Inlet Environment (BRIE) model, which modified and extended the combined model of Ashton and Lorenzo-Trueba (2015) to include inlet dynamics. The model simulated inlet formation (i.e., breaching) and cross-sectional area changes, and included alongshore sediment volume balancing between updrift and downdrift sides of the inlet. BRIE also included a stratigraphic model that keeps track of how sediment types (i.e., lagoonal, washover deposits, flood tidal shoals) are reworked over time (Nienhuis and Lorenzo-Trueba 2019).
Other models include that of Palalane and Larson (2020), ShorelineS (Roelvink et al. 2020), and IH-LANS (Alvarez-Cuesta et al. 2021). The Cascade model, which simulates shoreline changes for a region of barrier islands, was updated by Palalane and Larson (2020) to include XST components from Larson et al. (2016), which included overwash, beach and dune erosion, transport between the beach and offshore bar, and aeolian transport. The ShorelineS model, developed by Roelvink et al. (2020), models shoreline change, overwash, and includes the ability to split and merge barrier islands or spits. It is also planned for ShorelineS to be coupled with XBeach or Delft3D to simulate island and inlet migration in future work (Roelvink et al. 2020). Alvarez-Cuesta et al. (2021) developed the IH-LANS model which combines LST (using a modified version of CERC based on Hallermeier 1980) and XST (following Toimil et al. 2017), while also including specific formulations for engineering structures such as groins, seawalls, and breakwaters.
Models that Couple Shoreline Change and Shoreface Evolution
Although not limited to barrier island modeling, many long-term models now couple shoreline change and shoreface evolution models. One of the earliest examples of this approach was the 3DBeach model, published by Larson et al. (1990), which was a combination of SBEACH and GENESIS, and was capable of simulating dynamic profile features such as offshore bars.
Recently developed models incorporate equilibrium shoreline models as one aspect of their predictive capabilities. These models include CoSMoS-COAST (Vitousek et al. 2017), LX-Shore (Robinet et al. 2018), and COCOONED (Antolínez et al. 2019). CoSMoS-COAST combines the one-line model of Vitousek and Barnard (2015), the equilibrium model of Yates et al. (2009), a translation component similar to Bruun (1962), and a long-term residual shoreline trend following Long and Plant (2012). LX-Shore combines the wave model SWAN with LST (e.g., CERC, Kamphuis 1991) and XST (e.g., Davidson et al. 2013) formulations in a 2D horizontal grid, similar to the CEM model setup (Robinet et al. 2018). Lastly, the COCOONED model (Antolínez et al. 2019) couples a one-line approach similar to Vitousek and Barnard (2015), a cross-shore equilibrium model similar to Miller and Dean (2004), and the analytical dune erosion method of Kriebel and Dean (1993).
Notably, data assimilation techniques have been tried with many of these equilibrium shoreline models. Long and Plant (2012) were one of the first to use data assimilation for shoreline evolution predictions. They combined a modified version of the model of Yates et al. (2009), which predicts long-term and short-term trends of shoreline position, with a joint extended Kalman filter (eKF) assimilation approach that updates the model predictions based on shoreline position observations. Other models that have used Kalman filtering include CoSMos-COAST (Vitousek et al. 2017), ShoreFor (Ibaceta et al. 2020), and IH-LANS (Alvarez-Cuesta et al. 2021).
Extended Event-Scale Models
Another common modeling approach that combines XST and LST is the extension of multifaceted event-scale models for use in long-term simulations. Due to computational constraints, event-scale models have primarily been used to simulate short-term changes. However, recently they have also been employed and extended to predict long-term changes where computational burden is reduced through hydrodynamic averaging or lengthening the morphological time step.
Vemulakonda et al. (1988) were among the first to use this approach with the coastal inlet processes (CIP) model, which was originally developed to predict tidal inlet shoaling for ingress and egress of U.S. submarines. Wave and circulation models were coupled together with a sediment transport model, the latter of which required a user-defined time step that effectively extended the hydrodynamic conditions. Comparing model results to a year’s worth of navigation channel survey data, the model was shown to satisfactorily predict sediment transport rates (Vemulakonda et al. 1988).
A more recent and common approach is that of Lesser et al. (2004), who applied a morphological acceleration factor (morfac) within Delft3D to effectively lengthen the sediment transport time step for long-term simulations. Lesser et al. (2004) showed that using morfac in simplified cases did not cause the results to significantly deviate from the full solution. This approach was extended by Roelvink (2006), who proposed running multiple accelerated simulations in parallel for different tidal phases and using a weighted average of morphological change to update the bathymetry for the next time step.
Event-scale models are also used to model storm sequences and recovery periods between storms. Ranasinghe et al. (2012a) developed the probabilistic coastline recession (PCR) model, which generates 100-year sequences of storm events and employs the event-scale swash impact model of Larson et al. (2004a) (LEH04) to predict dune recession. The model also considered SLR projections and used a constant, empirically derived rate of dune recovery between storm events (Ranasinghe et al. 2012a). Long et al. (2020) developed a modeling framework for Breton Island, Louisiana, to assess restoration design alternatives that used XBeach to model the island’s response to successive storm events over a 15-year time period. Shoreface and bay-side erosion between storm events were not modeled explicitly, but were accounted for through manual manipulation of the prestorm profiles (Long et al. 2020).
Summary of Advancements and Limitations
The literature indicates that over the last fifty years, significant advancements have been made in long-term morphodynamic modeling of barrier systems. Again, model development has followed a rather natural progression—from the simplified to the complex. The intuition behind some of the earliest models (e.g., one-line and translation models) laid a foundation on which subsequent model development has been steadily built. More complex formulations have been developed to predict shoreface shape changes, rather than assuming a constant equilibrium profile. Additional processes have been added (e.g., overwash representations, changes in sediment supply) to more closely capture the underlying mechanics of barrier transgression. Models are also increasingly being developed to incorporate other sub-systems (e.g., the backbarrier marsh-lagoon system) that impact the long-term morphodynamics.
Yet there are still many limitations to be addressed, including (but not limited to) model validation, uncertainty characterization, and the incorporation of relevant processes and important factors. Although there is a wealth of satellite imagery available to coastal researchers, this dataset is limited both in the information it contains (i.e., primarily shoreline and marsh positions) and its temporal coverage for long-term model calibration and validation. This lack of long-term quantitative data is one likely reason why many long-term models have not been thoroughly validated. Other long-term models that were originally created to explore barrier island morphodynamics and develop new hypotheses—what Murray (2003) calls “exploratory models”—have largely remained as such and have not yet shifted toward the prediction of real systems. In addition, although testing model sensitivity is common practice, most models are not developed to explicitly consider input parameter uncertainty. Models typically receive averaged or representative input values and produce a single-value output rather than a statistical range of predictions. Another limitation, similar to event-scale modeling, is that most previous efforts have focused on evolution of the natural barrier system and have neglected anthropogenic impacts. Other relevant processes such as barrier subsidence, aeolian transport, backbarrier marsh growth/erosion, and factors that impact erosion and deposition such as vegetation type and density, have mostly been excluded from long-term models with only a few exceptions.
One modeling challenge that has persisted over time is the extrapolation of small-scale sediment transport predictions to large scale coastal behavior (LSCB)—a link which is certainly intuitive. However, the problem of uncertainty or error propagation, where uncertainty or error at the small-scale compounds over time result in imprecise or inaccurate predictions, has stifled this type of long-term modeling. De Vriend (1991a) indicates the extraordinary challenge of this unsolved problem saying, “…it must even be doubted whether models formulated at a small scale will ever be able to describe LSCB,” and reverently quips that “we may need another Ludwig Prandtl” before we have a good answer.
Research Gaps and Needs
Based on the advancements that have been made toward our Grand Challenge, and the limitations that persist in our modeling efforts, we have identified critical gaps and future research needs that might be addressed moving forward. The gaps and needs highlighted in the following are those we believe are most critical for making progress toward the Grand Challenge. We acknowledge, however, that other gaps and needs exist. The research gaps and needs may be generally categorized as follows: (1) observations, data availability and accessibility; (2) scientific understanding of relevant processes; and (3) modeling framework and approach. These categories are expounded as follows.
Observations, Data Availability, and Accessibility
One of the major limitations of our current modeling efforts is the availability of data. While technological advancements during the 20th century increased our ability to collect good data, the timing of these advancements means the quantity of long-term data for validation is sparse. However, event-scale data are not limited by time, but by the complexities and dangers associated with collecting perishable data before, during, and immediately following storm events. However, to improve our scientific understanding of the relevant processes and associated modeling efforts, we must overcome these data limitations so that we can ground truth our theories and formulations in observations. Herein, we discuss a few high-level issues regarding data acquisition and accessibility, while assuming that some methodological advancements for data collection and analysis will be required to further our understanding of the relevant processes discussed in the following section.
Long-term observations of coastal morphodynamics generally exist only at a limited number of well-monitored sites (e.g., Duck, NC, Larson and Kraus 1994; Torrey Pines, CA, Ludka et al. 2019; Ocean Beach, CA, Barnard et al. 2012; Fire Island, NY, Lentz and Hapke 2011; Narrabeen-Collaroy, Australia, Turner et al. 2016; Truc Vert, France, Castelle et al. 2020; Hasaki, Japan, Banno et al. 2020; South Holland, Netherlands, de Schipper et al. 2016), which are maintained by various government agencies and academic institutions. It is vital that these long-term monitoring efforts continue while new avenues of data at higher spatiotemporal resolutions are sought. As such, we must be diligent to make the most of available datasets, develop new ones, and make them broadly accessible. We must develop and promote centralized, open access databases (e.g., the Community Surface Dynamics Modeling System, CSDMS) that contain both open access models and collected data (e.g., the use of public archival in the National Science Foundation’s DesignSafe Rathje et al. 2017, or postevent field data Berman et al. 2020). Increasing the amount and quality of available data would also be useful for blind model comparisons, data assimilation, and machine learning applications.
One way to push toward increased dataset availability is to continue to capitalize on technologies that exist and are readily available. A perfect example of this is remote sensing data, such as publicly available satellite imagery (e.g., Luijendijk et al. 2018; Vos et al. 2019; Turner et al. 2021). We also expect that publicly accessible LiDaR datasets will become more widely available with continued advancements in drone technology (Shaw et al. 2019). It might also require us to creatively enlist the public’s help in data collection such as using public photos and photogrammetry (e.g., Harley et al. 2019). A second way to advance this initiative is by developing new data collection methods or technologies. Due to the perishable nature of prestorm and poststorm data and the uncertainties surrounding the timing and location of storm events, morphological data before, during, and after storm events is difficult to obtain. Certain efforts are underway to help coordinate, collect, and make available this perishable data, including the National Science Foundation’s NHERI RAPID Facility (Wartman et al. 2020; Berman et al. 2020) and the Nearshore Extreme Events Reconnaissance program (Raubenheimer 2020).
Scientific Understanding of Relevant Processes
Epistemic uncertainty and the exclusion of relevant factors are two important previously mentioned limitations. The epistemological issues discussed herein include both hydrodynamics and sediment transport, and the relevant factors discussed include vegetation dynamics and anthropogenic impacts.
Despite hydrodynamic simulation advancements, increased complexity in sediment transport formulations has not always translated to increased accuracy. Quoting from a study by Davies et al. (2002) in which multiple transport formulations were compared, Bosboom and Stive (2021) noted that most sediment transport predictions are only accurate within an order of magnitude, and that empirical calibration of these model formulations is still necessary in many cases. They also remarked that the simpler formulations are still often the best available ones. This indicates an obvious shortcoming in our ability to reproduce realistic hydrodynamic forcing conditions and to model the relationship between forcing and sediment transport. Aagaard and Hughes (2013) highlighted some of the latter shortcomings, stating that there is room for improvement in our quantitative understanding of bed load and suspended load transport, as well as our knowledge of which parameters (other than bed shear stress) can lead to better transport rate predictions. Notably, while such improvements would certainly lead to advancements in event-scale modeling efforts, the initial impact on long-term models would be minimal.
One of the greatest advancements in event-scale morphodynamic modeling in recent years was the inclusion of infragravity waves in the hydrodynamic calculations (Sherwood et al. 2022). While we still do not fully understand the mechanics of how these waves impact nearshore sediment transport (Aagaard and Kroon 2017), we now recognize their importance in predicting an event-scale morphodynamic response. Other factors such as the nonlinearity of incident waves, the interaction of incident and infragravity waves, and swash zone dynamics, including turbulence and boundary layer flows, may also prove to be key missing components in coupled hydrodynamics–sediment transport formulations that have a significant impact on event-scale morphodynamics. While these factors may be key missing components, the small scales needed to resolve some of these hydrodynamic and sediment transport processes would require computational resources that make such modeling practically infeasible at present. Continued computational advancements may help to alleviate such limitations.
In studying and developing formulations for event-scale processes such as overwash and breaching, it is important to consider all of the contributions to total inundation height, including tides, storm surge, and waves. The exclusion of one or more of these contributions can alter the total inundation height and corresponding morphological response. Furthermore, special consideration should be given to the timing of these contributions, as recent work has shown that time differences between the bay peak surge and ocean peak surge can lead to bay-side breaching (e.g., Shin 1996; McCall et al. 2010; Sherwood et al. 2014; Smallegan and Irish 2017).
Since data for event-scale morphodynamic response are sparse, future work should capitalize on previously published studies or available data from historical events (e.g., van Ormondt et al. 2020), which may yield additional insights into the nature of overwash and breaching. Moreover, since overwash and breach observations are difficult to obtain in the field, physical modeling that leverages advancements in data collection methods and instrumentation may also help us better understand and quantify these processes. Although these physical modeling studies would require careful consideration of potential scaling issues, we believe that valuable insights into the overwash and breaching processes remain to be gained from this method of study.
Another factor that may be prioritized for future studies is coastal vegetation. Currently, we have a general understanding of how vegetation impacts barrier morphodynamics (e.g., dune stabilization, subaerial accretion, increased flow roughness) and vice versa (e.g., van der Lugt et al. 2019); however, our quantitative understanding, and field-verification of that understanding, is further behind. Moving forward, beneficial research efforts would include the quantification of vegetation impact for parameters such as vegetation type, location, density, and hydrodynamic conditions for implementation in event-scale and long-term models. Recent studies (e.g., Ayat and Kobayashi 2015; Zinnert et al. 2019) indicated that this research is underway, and recent modeling studies (e.g., Passeri et al. 2018; van der Lugt et al. 2019) exemplify the initial stages of incorporating this information into event-scale morphodynamic analysis. Furthermore, with the U.S. Army Corps of Engineers’ recent release of international guidelines on the design and implementation of Natural and Nature-Based Features (NNBF) (Bridges et al. 2021), we expect future studies to quantify the performance of NNBF in various coastal environments.
Many coastal barriers are no longer representative of a natural environment as they are either developed or impacted by development and engineering structures on neighboring shorelines. Although many early studies and models sought to quantify the impact of engineering structures on littoral transport (e.g., one-line modeling of shoreline changes near groins), relatively few studies have quantitatively addressed the morphological impact of human development and other large-scale coastal restoration practices. In addition, we would benefit from better understanding how the coastal management process works holistically, including how policies are developed, how individual restoration decisions are made, and how studies which quantify anthropogenic impacts influence the management process, considering cultural, political, and socioeconomic differences across localities. This type of analysis has largely been absent in the barrier morphodynamics literature, with the exception of a few observational studies on the feedbacks between coastal protection and real estate values (e.g., Keeler et al. 2018), and modeling studies that consider the coupling of barrier morphodynamics with the incentives of developers and owners (e.g., McNamara and Werner 2008) and individuals in the coastal real estate market (e.g., McNamara and Keeler 2013). Moving forward, beneficial research topics would include understanding the quantitative morphodynamic response between developed and natural barrier systems (e.g., Rogers et al. 2015), and the incentives, behavior, and impacts of human agents in what is appropriately called a “coupled human–landscape” or “coupled natural–human” system (McNamara and Lazarus 2018; NASEM 2018).
Modeling Framework and Approach
There are several ways in which our modeling frameworks and overall approach may continue to improve to further research and achieve higher-confidence predictions. First, since modeling is inherently tied to the scientific understanding of the processes being studied, advancements in how those processes are understood must be regularly incorporated into the improvement of existing models and the development of new models. As research has naturally become more focused and specialized, many recent studies have been published related to specific components of barrier island morphodynamics (e.g., sediment transport between the inner shelf and active profile, beach–dune interactions, backbarrier marsh dynamics, etc.). Therefore it is critically important that holistic models of barrier morphodynamics incorporate the theory and formulations of more focused models.
Second, although some of the recently published long-term morphodynamic models included sensitivity analyses for various parameters, model results are still largely presented as single simulation output. Modeling efforts would benefit by increasingly employing ensemble approaches (e.g., Monte Carlo techniques) that consider input parameter uncertainty. Rather than producing a single output, a probabilistic range of results would be produced that can help characterize uncertainty in the model predictions (Vitousek et al. 2021). Such an approach lends itself not only to identifying expected values, but also to identifying extreme scenarios and the input parameter combinations that cause them. In addition, with the large number of models that have been developed, modelers may consider a multiple-model ensemble approach to evaluate the range of predictions across various models, as has been done with model comparison studies (e.g., Montaño et al. 2020). Such an approach would emulate the current practice for forecasting hurricanes and would also naturally facilitate model comparisons and identification of robust and accurate models.
Third, as we focus on expanding data accessibility and collection capabilities, we must be diligent to incorporate the available data. In addition to model validation, data may be used to train and/or reduce error in model predictions using machine learning and data assimilation methods, respectively. There are many ways in which machine learning may be employed in morphodynamic modeling to improve predictions and fine-tune model parameters for a specific site (Goldstein et al. 2019). Machine learning may also be employed to reduce computational burden. As models include relevant processes at smaller scales, the computational burden will naturally increase; however, machine learning techniques can serve to abstract those computationally expensive processes, effectively substituting a recognized or learned pattern for a more complex algorithm. One drawback to these powerful data-driven approaches is that it is possible to “over-train” a model with limited data, which effectively reduces its predictive capability for conditions that have yet to be observed. Despite the benefits and drawbacks of these methods, there are still relatively few models that explicitly incorporate them, suggesting there is still much room for model improvement.
Fourth, many models still focus only on parts of the barrier system, without considering all relevant processes. Such scientific focus up to this point was likely necessary to better understand specific system components; however, our current knowledge of important processes should lead to more complex, coupled, and fully representative models. For example, recent models (e.g., Walters et al. 2014; Lorenzo-Trueba and Mariotti 2017) have shown the importance of coupling the backbarrier marsh-lagoon system to barrier evolution models; however, there are still relatively few models that incorporate these as coupled systems. Barrier subsidence has received relatively little attention in the literature and has been incorporated into a minority of barrier evolution models (e.g., Rosati et al. 2006, 2010). Yet, from these few studies, we see that consolidation rates can significantly impact the future evolution of the system. The role of aeolian transport has also largely been neglected in barrier island evolution models. Although a large body of work exists regarding aeolian transport and its role in dune recovery (e.g., Brodie et al. 2017), few full-scale barrier evolution models have integrated this research. This may be the case, at least in part, because of the relatively recent focus on modeling storm sequences and poststorm beach and dune recovery (Eichentopf et al. 2019). However, as various studies have indicated the importance of these morphological components, modeling efforts would be most beneficial by driving toward the incorporation of all relevant processes.
Finally, anthropogenic influences, such as urban development and its associated infrastructure, have changed and will continue to change the way many of the fundamental processes discussed in this review affect barrier island morphology. This also includes coastal engineering infrastructure, which is often intended to reduce inundation and erosion, or to support recreational and commercial navigation. Thus, modeling paradigms shifted toward representing barrier islands as coupled human–natural systems would provide important insights (McNamara and Lazarus 2018). Modeling frameworks that included anthropogenic impacts such as the effects of human agents (e.g., McNamara and Werner 2008), urban development (e.g., Rogers et al. 2015), and coastal restoration practices (e.g., Long et al. 2020), would help us explore and evaluate their impacts which would be useful in coastal planning.
Summary
In closing, future research and development in the area of morphodynamic modeling of coastal barrier systems would benefit by leveraging existing and new datasets, advancements in observation technologies, and emerging data science approaches to better characterize morphological response and its uncertainty. Continuing the research community shift toward open access models and data would facilitate more rapid advancement in this area. Scientific advances are most needed in understanding anthropogenic and ecological influences on barrier morphological change. Also essential is advancing scientific understanding of observed morphological phenomena and the underlying sediment transport processes, including the coupling between a barrier and its sub-systems. Such advancements will bring us closer to achieving the overarching goal of high-confidence predictions of barrier system morphodynamics in multiple spatiotemporal dimensions.
Data Availability Statement
No data, models, or code were generated or used during the study.
Acknowledgments
This material is based upon work that is primarily supported by the U.S. Army Corps of Engineers through the U.S. Coastal Research Program (under Grant No. W912HZ-20-2-0005) and partially supported by the National Science Foundation (under Grant No. 1735139). Any opinions, findings, and conclusions or recommendations expressed in this material are those of the authors and do not necessarily reflect the views of these organizations. This publication was also prepared in part by Steven Hoagland using Federal funds under award NA18OAR4170083, Virginia Sea Grant College Program Project R/72155T, from the National Oceanic and Atmospheric Administration’s (NOAA) National Sea Grant College Program, U.S. Department of Commerce. The statements, findings, conclusions, and recommendations are those of the author(s) and do not necessarily reflect the views of Virginia Sea Grant, NOAA, or the U.S. Department of Commerce.
Disclaimer
Disclaimer for nonendorsement of commercial products and services: Any use of trade, firm, or product names is for descriptive purposes only and does not imply endorsement by the U.S. Government.
References
Aagaard, T., and M. Hughes. 2013. “Sediment transport.” Chap. 10.4 in Vol. 10 of Treatise on geomorphology, edited by J. Shroder and D. Sherman, 74–105. San Diego: Academic Press.
Aagaard, T., and A. Kroon. 2017. “Sediment transport under storm conditions on sandy beaches.” Chap. 3 in Coastal storms: Processes and impacts, edited by P. Ciavola and G. Coco, 44–63. Hoboken, NJ: John Wiley & Sons.
Alfageme, S., and R. Cañizares. 2005. “Process-based morphological modeling of a restored barrier island: Whiskey Island, Louisiana, USA.” In Proc., 5th Int. Conf. on Coastal Dynamics, 1–11. Reston, VA: ASCE.
Alfageme, S. R., M. Khondker, and R. Canizares. 2007. “Breach stability and growth analysis using a morphological model.” In Proc., 6th Int. Symp. on Coastal Engineering and Science of Coastal Sediment Processes, 2025–2036. Reston, VA: ASCE.
Alvarez-Cuesta, M., A. Toimil, and I. J. Losada. 2021. “Modelling long-term shoreline evolution in highly anthropized coastal areas. Part 1: Model description and validation.” Coastal Eng. 169. 103960. https://doi.org/10.1016/j.coastaleng.2021.103960.
Antolínez, J. A., F. J. Méndez, D. Anderson, P. Ruggiero, and G. M. Kaminsky. 2019. “Predicting climate-driven coastlines with a simple and efficient multiscale model.” J. Geophys. Res.: Earth Surf. 124 (6): 1596–1624. https://doi.org/10.1029/2018JF004790.
Armon, J. W., and S. B. McCann. 1979. “Morphology and landward sediment transfer in a transgressive barrier island system, Southern Gulf of St. Lawrence, Canada.” Mar. Geol. 31 (3–4): 333–344. https://doi.org/10.1016/0025-3227(79)90041-0.
Ashton, A. D., and J. Lorenzo-Trueba. 2015. “Complex responses of barriers to sea-level rise emerging from a model of alongshore-coupled dynamic profile evolution.” In Vol. 7 of Proc., Coastal Sediments 2015, 1–7. Singapore: World Scientific.
Ashton, A. D., and A. B. Murray. 2006. “High-angle wave instability and emergent shoreline shapes: 1. Modeling of sand waves, flying spits, and capes.” J. Geophys. Res.: Earth Surf. 111 (4): 1–19.
Ashton, A. D., A. B. Murray, and O. Amoult. 2001. “Formation of coastline features by large-scale instabilities induced by high-angle waves.” Nature 414 (6861): 296–300. https://doi.org/10.1038/35104541.
Atkinson, A. L., T. E. Baldock, F. Birrien, D. P. Callaghan, P. Nielsen, T. Beuzen, I. L. Turner, C. E. Blenkinsopp, and R. Ranasinghe. 2018. “Laboratory investigation of the Bruun Rule and beach response to sea level rise.” Coastal Eng. 136 (2017): 183–202. https://doi.org/10.1016/j.coastaleng.2018.03.003.
Ayat, B., and N. Kobayashi. 2015. “Vertical cylinder density and toppling effects on dune erosion and overwash.” J. Waterway, Port, Coastal, Ocean Eng. 141 (1): 04014026. https://doi.org/10.1061/(ASCE)WW.1943-5460.0000264.
Bagnold, R. A. 1963. “Mechanics of marine sedimentation.” In Vol. 3 of The Sea, Ideas and Observations on Progress in the Study of the Sea – The Earth Beneath the Sea: History, edited by M. N. Hill, 507–528. New York: John Wiley & Sons.
Bagnold, R. A. 1966. “An approach to the sediment transport problem from general physics.” Geological Survey Professional Paper 422–I. Washington, DC: United States Government Printing Office.
Bakker, W. 1968. “The dynamics of a coast with a groyne system.” In Proc., 11th Int. Conf. on Coastal Engineering, 492–517. Reston, VA: ASCE.
Baldock, T. E., M. G. Hughes, K. Day, and J. Louys. 2005. “Swash overtopping and sediment overwash on a truncated beach.” Coastal Eng. 52 (7): 633–645. https://doi.org/10.1016/j.coastaleng.2005.04.002.
Banno, M., S. Nakamura, T. Kosako, Y. Nakagawa, S. I. Yanagishima, and Y. Kuriyama. 2020. “Long-term observations of beach variability at Hasaki, Japan.” J. Mar. Sci. Eng. 8 (11): 1–17. https://doi.org/10.3390/jmse8110871.
Barnard, P. L., J. E. Hansen, and L. H. Erikson. 2012. “Synthesis study of an erosion hot spot, Ocean Beach, California.” J. Coastal Res. 28 (4): 903–922. https://doi.org/10.2112/JCOASTRES-D-11-00212.1.
Basco, D. R., and C. S. Shin. 1999. “A one-dimensional numerical model for storm-breaching of barrier islands.” J. Coastal Res. 15 (1): 241–260.
Belknap, D. F., and J. C. Kraft. 1985. “Influence of antecedent geology on stratigraphic preservation potential and evolution of Delaware’s barrier systems.” Mar. Geol. 63 (1–4): 235–262. https://doi.org/10.1016/0025-3227(85)90085-4.
Berman, J. W., et al. 2020. “Natural hazards reconnaissance with the NHERI rapid facility.” Front. Built Environ. 6 (Nov): 1–16.
Bosboom, J., and M. J. F. Stive. 2021. Coastal dynamics. Delft, The Netherlands: Delft Univ. of Technology.
Brenner, O. T., L. J. Moore, and A. B. Murray. 2015. “The complex influences of back-barrier deposition, substrate slope and underlying stratigraphy in barrier island response to sea-level rise: Insights from the Virginia Barrier Islands, Mid-Atlantic Bight, USA.” Geomorphology 246: 334–350. https://doi.org/10.1016/j.geomorph.2015.06.014.
Bridges, T., J. King, J. Simm, M. Beck, G. Collins, Q. Lodder, and R. Mohan. 2021. International guidelines on natural and nature-based features for flood risk management. Rep. No. Washington, DC: US Army Corps of Engineers.
Bridges, T. S., R. Henn, S. Komlos, D. Scerno, T. Wamsley, and K. White. 2013. Coastal risk reduction and resilience. Washington, DC: United States Army Corps of Engineers, Civil Works Directorate.
Brodie, K. L., M. L. Palmsten, and N. J. Spore. 2017. Coastal foredune evolution, Part 1: Environmental factors & forcing processes affecting morphological evolution. Technical Rep. Erdc/Chl Chetn-Ii-56. Washington, DC: US Army Corps of Engineers.
Bruun, P. 1962. “Sea level rise as a cause of shore erosion.” J. Waterways Harbors Div. 88 (1): 117–130. https://doi.org/10.1061/JWHEAU.0000252.
Bruun, P. 1983. “Review of conditions for uses of the Bruun rule of erosion.” Coastal Eng. 7 (1): 77–89. https://doi.org/10.1016/0378-3839(83)90028-5.
Buijsman, M. C. 1997. “The impact of gas extraction and sea level rise on the morphology of the Wadden Sea: Extension and application of the model ASMITA.” Rep. No. H 3099.30. Netherlands: Center for Coastal Research.
Callaghan, D. P., R. Ranasinghe, and D. Roelvink. 2013. “Probabilistic estimation of storm erosion using analytical, semi-empirical, and process based storm erosion models.” Coastal Eng. 82: 64–75. https://doi.org/10.1016/j.coastaleng.2013.08.007.
Cañizares, R., and J. L. Irish. 2008. “Simulation of storm-induced barrier island morphodynamics and flooding.” Coastal Eng. 55 (12): 1089–1101. https://doi.org/10.1016/j.coastaleng.2008.04.006.
Castelle, B., S. Bujan, V. Marieu, and S. Ferreira. 2020. “16 years of topographic surveys of rip-channelled high-energy meso-macrotidal sandy beach.” Sci. Data 7 (1): 1–9. https://doi.org/10.1038/s41597-020-00750-5.
Chardón-Maldonado, P., J. C. Pintado-Pati no, and J. A. Puleo. 2016. “Advances in swash-zone research: Small-scale hydrodynamic and sediment transport processes.” Coastal Eng. 115: 8–25. https://doi.org/10.1016/j.coastaleng.2015.10.008.
Cho, M., H.-D. Yoon, K. Do, S. Son, and I.-H. Kim. 2019. “Comparative study on the numerical simulation of bathymetric changes under storm condition.” J. Coastal Res. 91 (sp1): 106. https://doi.org/10.2112/SI91-022.1.
Ciavola, P., and G. Coco, eds. 2017. Coastal storms. Chichester, UK: John Wiley & Sons.
Cleary, W. J., and P. E. Hosier. 1979. “Geomorphology, washover history, and inlet zonation: Cape lookout, North Carolina to Bird Island, North Carolina.” In Barrier islands: From the Gulf of St. Lawrence to the Gulf of Mexico, edited by S. P. Leatherman. Cambridge, MA: Academic Press.
Conley, D. C. 1999. “Observations on the impact of a developing inlet in a bar built estuary.” Cont. Shelf Res. 19 (13): 1733–1754. https://doi.org/10.1016/S0278-4343(99)00035-7.
Cowell, P., P. Roy, and R. Jones. 1992. “Shoreface translation model: Computer simulation of coastal-sandbody response to sea level rise.” Math. Comput. Simul. 33 (5–6): 603–608. https://doi.org/10.1016/0378-4754(92)90158-D.
Cowell, P., P. S. Roy, and R. A. Jones. 1995. “Simulation of large-scale coastal change using a morphological behaviour model.” Mar. Geol. 126 (1–4): 45–61. https://doi.org/10.1016/0025-3227(95)00065-7.
Cowell, P. J., M. J. Stive, A. W. Niedoroda, H. J. De Vriend, D. J. Swift, G. M. Kaminsky, and M. Capobianco. 2003a. “The coastal-tract (Part 1): A conceptual approach to aggregated modeling of low-order coastal change.” J. Coastal Res. 19 (4): 812–827.
Cowell, P. J., et al. 2003b. “The coastal-tract (Part 2): Applications of aggregated modeling of lower-order coastal change.” J. Coastal Res. 19 (4): 828–848.
Cowell, P. J., B. G. Thom, R. A. Jones, C. H. Everts, and D. Simanovic. 2006. “Management of uncertainty in predicting climate-change impacts on beaches.” J. Coastal Res. 22 (1): 232–245. https://doi.org/10.2112/05A-0018.1.
D’anna, M., D. Idier, B. Castelle, S. Vitousek, and G. Le Cozannet. 2021. “Reinterpreting the Bruun rule in the context of equilibrium shoreline models.” J. Mar. Sci. Eng. 9 (9): 974. https://doi.org/10.3390/jmse9090974.
Davidson, M. A., K. D. Splinter, and I. L. Turner. 2013. “A simple equilibrium model for predicting shoreline change.” Coastal Eng. 73: 191–202. https://doi.org/10.1016/j.coastaleng.2012.11.002.
Davidson, M. A., I. L. Turner, K. D. Splinter, and M. D. Harley. 2017. “Annual prediction of shoreline erosion and subsequent recovery.” Coastal Eng. 130 (Oct): 14–25. https://doi.org/10.1016/j.coastaleng.2017.09.008.
Davies, A. G., L. C. Van Rijn, J. S. Damgaard, J. Van De Graaff, and J. S. Ribberink. 2002. “Intercomparison of research and practical sand transport models.” Coastal Eng. 46 (1): 1–23. https://doi.org/10.1016/S0378-3839(02)00042-X.
De Goede, E. D. 2020. “Historical overview of 2D and 3D hydrodynamic modelling of shallow water flows in the Netherlands.” Ocean Dyn. 70 (4): 521–539. https://doi.org/10.1007/s10236-019-01336-5.
de Schipper, M. A., S. de Vries, G. Ruessink, R. C. de Zeeuw, J. Rutten, C. van Gelder-Maas, and M. J. Stive. 2016. “Initial spreading of a mega feeder nourishment: Observations of the Sand Engine pilot project.” Coastal Eng. 111: 23–38. https://doi.org/10.1016/j.coastaleng.2015.10.011.
De Vet, P. 2014. “Modelling sediment transport and morphology during overwash and breaching events.” M.Sc. thesis, Dept. of Civil Engineering, Delft Univ. of Technology.
De Vriend, H. J. 1991a. “Mathematical modelling and large-scale coastal behavior – Part 2: Predictive Models.” J. Hydraul. Res. 29 (6): 741–753. https://doi.org/10.1080/00221689109498956.
De Vriend, H. J. 1991b. “Mathematical modelling and large-scale coastal behaviour - Part 1: Physical Processes.” J. Hydraul. Res. 29 (6): 727–740. https://doi.org/10.1080/00221689109498955.
de Vriend, H. J., M. Capobianco, T. Chesher, H. E. de Swart, B. Latteux, and M. J. Stive. 1993. “Approaches to long-term modelling of coastal morphology: A review.” Coastal Eng. 21 (1–3): 225–269. https://doi.org/10.1016/0378-3839(93)90051-9.
Dean, R. G. 1991. “Equilibrium beach profiles: characteristics and applications.” J. Coastal Res. 7 (1): 53–84.
Dean, R. G., and R. A. Dalrymple. 2002. Coastal processes with engineering applications. Cambridge, UK: Cambridge University Press.
Dean, R. G., and J. R. Houston. 2016. “Determining shoreline response to sea level rise.” Coastal Eng. 114: 1–8. https://doi.org/10.1016/j.coastaleng.2016.03.009.
Dean, R. G., and E. Maurmeyer. 1983. “Models for beach profile response.” Chap. 7 in Handbook of coastal processes and erosion, edited by P. D. Komar. Boca Raton, FL: CRC Press.
Deltares. 2021. “UNIBEST-CL+.” Accessed October 1, 2021. https://www.deltares.nl/en/software-and-data/products/unibest-cl.
Dillon, W. 1970. “Submergence effects on a Rhode Island Barrier and Lagoon and inferences on migration of barriers.” J. Geol. 78 (1): 94–106. https://doi.org/10.1086/627490.
Dissanayake, P., J. Brown, and H. Karunarathna. 2015. “Impacts of storm chronology on the morphological changes of the Formby beach and dune system, UK.” Nat. Hazards Earth Syst. Sci. 15 (7): 1533–1543. https://doi.org/10.5194/nhess-15-1533-2015.
Dolan, R., and H. Lins. 1986. The outer banks of North Carolina. US Geological Survey Professional Paper 1177-B.
Donnelly, C., N. Kraus, and M. Larson. 2006. “State of knowledge on measurement and modeling of coastal overwash.” J. Coastal Res. 22 (4): 965–991. https://doi.org/10.2112/04-0431.1.
Donnelly, C., M. Larson, and H. Hanson. 2009. “A numerical model of coastal overwash.” Proc. Inst. Civ. Eng. Marit. Eng. 162 (3): 105–114. https://doi.org/10.1680/maen.2009.162.3.105.
Donnelly, C., R. Ranasinghe, and M. Larson. 2005. “Numerical modeling of beach profile change caused by overwash.” In Proc., 5th Coastal Dynamics Int. Conf., 1–15. Reston, VA: ASCE.
Dubois, R. N. 1993. “Discussion of Orrin Pilkey, Robert S. Young, Stanley R. Riggs, A. W. Sam Smith, Huiyan Wu and Walter D. Pilkey, 1993. The concept of shoreface profile of equilibrium: A critical review.” J. Coastal Res. 9 (4): 28–31.
Edelman, T. 1968. “Dune erosion during storm conditions.” In Proc., 11th Int. Conf. on Coastal Engineering, 719–722. Reston, VA: ASCE.
Edelman, T. 1972. “Dune erosion during storm conditions.” In Proc., 13th Int. Conf. on Coastal Engineering, 1305–1311. Reston, VA: ASCE.
Eichentopf, S., H. Karunarathna, and J. M. Alsina. 2019. “Morphodynamics of sandy beaches under the influence of storm sequences: Current research status and future needs.” Water Sci. Eng. 12 (3): 221–234. https://doi.org/10.1016/j.wse.2019.09.007.
Elsayed, S. M., and H. Oumeraci. 2017. “Effect of beach slope and grain-stabilization on coastal sediment transport: An attempt to overcome the erosion overestimation by XBeach.” Coastal Eng. 121 (Jun): 179–196. https://doi.org/10.1016/j.coastaleng.2016.12.009.
ESA (The European Space Agency). 2021. “Sentinel-2 satellite imagery (courtesy of the US Geological Survey).” Accessed October 1, 2021. https://sentinel.esa.int/web/sentinel/missions/sentinel-2.
Everts, C. H. 1985. “Sea level rise effects on shoreline position.” J. Waterw. Port, Coast. Ocean Eng. 111 (6): 985–999.
Everts, C. H. 1987. “Continental shelf evolution in response to a rise in sea level.” In Sea-Level Fluctuations and Coastal Evolution, 49–57. Broken Arrow, OK: Society of Economic Paleontologists and Mineralogists.
Fisher, J., and M. Overton. 1984. “Numerical model for dune erosion due to wave uprush.” In Proc., 19th Int. Conf. on Coastal Engineering, edited by B. L. Edge, 1553–1558. Reston, VA: ASCE.
Fisher, J. J., and E. J. Simpson. 1979. “Washover and tidal sedimentation rates as environmental fators in development of a transgressive barrier shoreline.” In Barrier Islands: From the Gulf of St. Lawrence to the Gulf of Mexico, edited by S. P. Leatherman, 127–148. Cambridge, MA: Academic Press.
Fisher, J. S., M. F. Overton, and T. Chisholm. 1987. “Field measurements of dune erosion.” In Vol. 2 of Proc., 20th Int. Conf. on Coastal Engineering, 1107–1115. Reston, VA: ASCE.
Frey, A. E., K. J. Connell, H. Hanson, M. Larson, R. C. Thomas, S. Munger, and A. Zundel. 2012. GenCade version 1 model theory and user’s guide. TR-12-25. Vicksburg, MS: Coastal and Hydraulics Laboratory - U.S. Army Corps of Engineers.
Gerwing, T. G., E. Plate, J. Kidd, J. Sinclair, C. W. Burns, S. Johnson, S. Roias, C. McCulloch, and R. C. Bocking. 2020. “Immediate response of fish communities and water chemistry to causeway breaching and bridge installation in the Kaouk River estuary, British Columbia, Canada.” Restor. Ecol. 28 (3): 623–631. https://doi.org/10.1111/rec.v28.3.
Godfrey, P. J. 1970. Oceanic overwash and its ecological implications on the Outer Banks of North Carolina. Rep. No. Washington, DC: Office of Natural Science Studies, National Parks Service.
Goldstein, E. B., G. Coco, and N. G. Plant. 2019. “A review of machine learning applications to coastal sediment transport and morphodynamics.” Earth Sci. Rev. 194 (April): 97–108. https://doi.org/10.1016/j.earscirev.2019.04.022.
Gravens, M. B., R. M. Males, and D. A. Moser. 2007. “Beach-FX: Monte Carlo life-cycle simulation model for estimating shore protection project evolution and cost benefit analyses.” Shore Beach 75 (1): 12–19.
Grzegorzewski, A. S., M. A. Cialone, and T. V. Wamsley. 2011. “Interaction of Barrier Islands and Storms: Implications for flood risk reduction in Louisiana and Mississippi.” J. Coastal Res. 59: 156–164. https://doi.org/10.2112/SI59-016.1.
Grzegorzewski, A. S., B. D. Johnson, T. V. Wamsley, and J. D. Rosati. 2013. “Sediment transport and morphology modeling of Ship Island, Mississippi, USA, during storm events.” In Proc., Coastal Dynamics 2013. 1505–1516. Arcachon, France: Coastal Dynamics.
Gutierrez, B. T., S. J. Williams, and E. R. Thieler. 2007. Potential for shoreline changes due to sea-level rise along the US mid-Atlantic region. US Geological Survey Open-File Report 2007–1278. https://pubs.usgs.gov/of/2007/1278.
Hallermeier, R. J. 1980. “A profile zonation for seasonal sand beaches from wave climate.” Coastal Eng. 4 (C): 253–277. https://doi.org/10.1016/0378-3839(80)90022-8.
Halsey, S. D. 1979. “Nexus: New model of barrier island development.” In Barrier Islands: From the Gulf of St. Lawrence to the Gulf of Mexico, edited by S. P. Leatherman. Cambridge, MA: Academic Press.
Hanson, H., and N. C. Kraus. 1989. GENESIS: Generalized model for simulating shoreline change. Technical Rep. CERC-89-19. Washington, DC: US Army Corps of Engineers.
Harley, M. D., M. A. Kinsela, E. Sánchez-García, and K. Vos. 2019. “Shoreline change mapping using crowd-sourced smartphone images.” Coastal Eng. 150: 175–189. https://doi.org/10.1016/j.coastaleng.2019.04.003.
Harter, C., and J. Figlus. 2017. “Numerical modeling of the morphodynamic response of a low-lying barrier island beach and foredune system inundated during Hurricane Ike using XBeach and CSHORE.” Coastal Eng. 120 (Apr): 64–74. https://doi.org/10.1016/j.coastaleng.2016.11.005.
Hoonhout, B. 2009. “Dune erosion along curved coastlines.” M.Sc. thesis, Dept. of Hydraulic Engineering, Delft Univ. of Technology.
Houston, J. R. 1996. “Discussion of: Young, R.S.; Pilkey, O.H.; Bush, D.M.; and Thieler, E.R. A discussion of the generalized model for simulating shoreline change (GENESIS), Journal of Coastal Research 11(3), 875–886.” J. Coastal Res. 12 (4): 1038–1043.
Hoyt, J. 1969. “Chenier versus barrier, genetic and stratigraphic distinction.” Am. Assoc. Pet. Geol. Bull. 53 (2): 299–306. https://doi.org/10.1306/5D25C60D-16C1-11D7-8645000102C1865D.
Ibaceta, R., K. D. Splinter, M. D. Harley, and I. L. Turner. 2020. “Enhanced coastal shoreline modeling using an ensemble Kalman filter to include nonstationarity in future wave climates.” Geophys. Res. Lett. 47 (22): 1–12. https://doi.org/10.1029/2020GL090724.
Jiménez, J. A., and A. Sánchez-Arcilla. 2004. “A long-term (decadal scale) evolution model for microtidal barrier systems.” Coastal Eng. 51 (8–9): 749–764. https://doi.org/10.1016/j.coastaleng.2004.07.007.
Johnson, B. D., N. Kobayashi, and M. B. Gravens. 2012. Cross-shore numerical model CSHORE for waves, currents, sediment transport and beach profile evolution. Rep. No. ERDC/CHL TR 12-22. Washington, DC: US Army Corps of Engineers.
Johnson, C. L., Q. Chen, C. E. Ozdemir, K. Xu, R. McCall, and K. Nederhoff. 2021. “Morphodynamic modeling of a low-lying barrier subject to hurricane forcing: The role of backbarrier wetlands.” Coastal Eng. 167 (Apr): 103886. https://doi.org/10.1016/j.coastaleng.2021.103886.
Johnson, D. W. 1919. Shore processes and shoreline development. Hoboken, NJ: John Wiley & Sons.
Kamphuis, J. W. 1991. “Alongshore sediment transport rate distribution.” J. Waterway, Port, Coastal, Ocean Eng. 117 (6): 170–183.
Kaveh, K., M. Reisenbüchler, S. Lamichhane, T. Liepert, N. D. Nguyen, M. D. Bui, and P. Rutschmann. 2019. “A comparative study of comprehensive modeling systems for sediment transport in a curved open channel.” Water 11 (9): 1779. https://doi.org/10.3390/w11091779.
Keeler, A. G., D. E. McNamara, and J. L. Irish. 2018. “Responding to sea level rise: Does short-term risk reduction inhibit successful long-term adaptation?” Earth’s Future 6 (4): 618–621. https://doi.org/10.1002/eft2.v6.4.
Kim, S.-C., R. Styles, J. Rosati, Y. Ding, and R. Permenter. 2020. A comparison of GenCade, Pelnard-Considere, and LITPACK. Rep. No. Washington, DC: US Army Corps of Engineers.
Kobayashi, N. 1987. “Analytical solution for dune erosion by storms.” J. Waterway, Port, Coastal, Ocean Eng. 113 (4): 401–418. https://doi.org/10.1061/(ASCE)0733-950X(1987)113:4(401).
Kobayashi, N. 2016. “Coastal sediment transport modeling for engineering applications.” J. Waterway, Port, Coastal, Ocean Eng. 142 (6): 03116001. https://doi.org/10.1061/(ASCE)WW.1943-5460.0000347.
Kobayashi, N., and A. Farhadzadeh. 2008. “Cross-shore numerical model CSHORE for waves, currents, sediment transport and beach profile evolution.” Report No. CACR-08-01. Center for Applied Coastal Research, Ocean Engineering Laboratory. Newark, DE: Univ. of Delaware.
Kobayashi, N., A. Farhadzadeh, J. Melby, B. D. Johnson, and M. B. Gravens. 2010. “Wave overtopping of levees and overwash of dunes.” J. Coastal Res. 26 (5): 888–900. https://doi.org/10.2112/JCOASTRES-D-09-00034.1.
Kobayashi, N., A. Payo, and L. Schmied. 2008. “Cross-shore suspended sand and bed load transport on beaches.” J. Geophys. Res.: Oceans 113 (7): 1–17.
Kobayashi, N., Y. Tega, and M. W. Hancock. 1996. “Wave reflection and overwash of dunes.” J. Waterway, Port, Coastal, Ocean Eng. 122 (3): 150–153. https://doi.org/10.1061/(ASCE)0733-950X(1996)122:3(150).
Komar, P. D., W. McDougal, J. Marra, and P. Ruggiero. 1999. “The rational analysis of setback distances: Applications to the Oregon Coast.” Shore Beach 67 (1): 41–49.
Komar, P. D., and J. R. Moore. 1983. Handbook of coastal processes and erosion. Boca Raton, FL: CRC Press.
Kraus, N., A. Militello, and G. Todoroff. 2002. “Barrier beaching processes and barrier spit breach, Stone Lagoon, California.” Shore Beach 70 (4): 21–28.
Kraus, N. C. 2003. “Analytical model of incipient breaching of coastal barriers.” Coastal Eng. J. 45 (4): 511–531. https://doi.org/10.1142/S057856340300097X.
Kraus, N. C., and K. Hayashi. 2005. “Numerical morphologic model of barrier island breaching.” In Proc., Coastal Engineering 2004, 2120–2132. Singapore: World Scientific Publishing Company.
Kraus, N. C., K. Patsch, and S. Munger. 2008. “Barrier beach breaching from the lagoon side, with reference to Northern California.” Shore Beach 76 (2): 33–43.
Kraus, N. C., and T. V. Wamsley. 2003. Coastal barrier breaching, Part 1: Overview of breaching processes. Washington, DC: US Army Corps of Engineers.
Kriebel, D. L., and R. G. Dean. 1985. “Numerical simulation of time-dependent beach and dune erosion.” Coastal Eng. 9 (3): 221–245. https://doi.org/10.1016/0378-3839(85)90009-2.
Kriebel, D. L., and R. G. Dean. 1993. “Convolution method for time-dependent beach-profile response.” J. Waterway, Port, Coastal, Ocean Eng. 119 (2): 204–226. https://doi.org/10.1061/(ASCE)0733-950X(1993)119:2(204).
Larson, M., C. Donnelly, J. A. Jiménez, and H. Hanson. 2009. “Analytical model of beach erosion and overwash during storms.” Proc. Inst. Civ. Eng. Marit. Eng. 162 (3): 115–125. https://doi.org/10.1680/maen.2009.162.3.115.
Larson, M., L. Erikson, and H. Hanson. 2004a. “An analytical model to predict dune erosion due to wave impact.” Coastal Eng. 51 (8–9): 675–696. https://doi.org/10.1016/j.coastaleng.2004.07.003.
Larson, M., H. Hanson, and N. C. Kraus. 1987. Analytical solutions of the one-line model of shoreline change. Technical Rep. CERC-87-15. Vicksburg, MI: US Army Engineer Waterways Experiment Station.
Larson, M., and N. Kraus. 1989. SBEACH: Numerical model for simulating storm-induced beach change. Technical Rep. CERC-89-9. Vicksburg, MI: US Army Engineer Waterways Experiment Station.
Larson, M., and N. C. Kraus. 1994. “Temporal and spatial scales of beach profile change, Duck, North Carolina.” Mar. Geol. 117 (1–4): 75–94. https://doi.org/10.1016/0025-3227(94)90007-8.
Larson, M., N. C. Kraus, and H. Hanson. 1990. “Decoupled numerical model of three-dimensional beach change.” In Proc., 22nd Int. Conf. on Coastal Engineering, Delft, 2173–2185. Reston, VA: ASCE.
Larson, M., N. C. Kraus, and H. Hanson. 2002. “Simulation of regional longshore sediment transport and coastal evolution – the “Cascade” model.” In Proc., Coastal Engineering 2002, 2612–2624. Singapore: World Scientific Publishing Company.
Larson, M., J. Palalane, C. Fredriksson, and H. Hanson. 2016. “Simulating cross-shore material exchange at decadal scale. Theory and model component validation.” Coastal Eng. 116: 57–66. https://doi.org/10.1016/j.coastaleng.2016.05.009.
Larson, M., R. Wise, and N. C. Kraus. 2004b. Coastal Overwash, Part 2: Upgrade to SBEACH. Coastal and Hydraulics Engineering Technical Note ERDC/CHL CHETN-XIV-14. Vicksburg, MS: US Army Engineer Research and Development Center, Coastal and Hydraulics Laboratory.
Latteux, B. 1995. “Techniques for long-term morphological simulation under tidal action.” Mar. Geol. 126 (1–4): 129–141. https://doi.org/10.1016/0025-3227(95)00069-B.
Leatherman, S. P. 1976. “Quantification of Overwash Processes.” Ph.D. thesis, Dept. of Environmental Sciences, Univ. of Virginia.
Leatherman, S. P. 1979. “Migration of Assateague Island, Maryland, by inlet and overwash processes.” Geology 7: 104–107. https://doi.org/10.1130/0091-7613(1979)7%A1104:MOAIMB%BF2.0.CO;2.
Leatherman, S. P. 1983. “Barrier dynamics and landward migration with Holocene sea-level rise.” Nature 301 (5899): 415–417. https://doi.org/10.1038/301415a0.
Leatherman, S. P. 1987. “Annotated chronological bibliography of barrier island migration.” J. Coastal Res. 3 (1): 1–14.
Lentz, E. E., and C. J. Hapke. 2011. “Geologic framework influences on the geomorphology of an anthropogenically modified barrier island: Assessment of dune/beach changes at Fire Island, New York.” Geomorphology 126 (1–2): 82–96. https://doi.org/10.1016/j.geomorph.2010.10.032.
Lesser, G. R., J. A. Roelvink, J. A. van Kester, and G. S. Stelling. 2004. “Development and validation of a three-dimensional morphological model.” Coastal Eng. 51 (8–9): 883–915. https://doi.org/10.1016/j.coastaleng.2004.07.014.
List, J. H., A. S. Farris, and C. Sullivan. 2006. “Reversing storm hotspots on sandy beaches: Spatial and temporal characteristics.” Mar. Geol. 226 (3–4): 261–279. https://doi.org/10.1016/j.margeo.2005.10.003.
Long, J., P. S. Dalyander, M. Poff, B. Spears, B. Borne, D. Thompson, R. Mickey, S. Dartez, and G. Grandy. 2020. “Event and decadal-scale modeling of barrier island restoration designs for decision support.” Shore Beach 88 (1): 49–57. https://doi.org/10.34237/01.
Long, J. W., and N. G. Plant. 2012. “Extended Kalman Filter framework for forecasting shoreline evolution.” Geophys. Res. Lett. 39 (13): 1–6. https://doi.org/10.1029/2012GL052180.
Lorenzo-Trueba, J., and A. D. Ashton. 2014. “Rollover, drowning, and discontinuous retreat: Distrinct modes of barrier response to sea-level rise arising from a simple morphodynamic model.” J. Geophys. Res.: Earth Surf. 119 (4): 779–801. https://doi.org/10.1002/2013JF002941.
Lorenzo-Trueba, J., and G. Mariotti. 2017. “Chasing boundaries and cascade effects in a coupled barrier-marsh-lagoon system.” Geomorphology 290: 153–163. https://doi.org/10.1016/j.geomorph.2017.04.019.
Ludka, B. C., et al. 2019. “Sixteen years of bathymetry and waves at San Diego beaches.” Sci. Data 6 (1): 1–13. https://doi.org/10.1038/s41597-019-0167-6.
Luijendijk, A., G. Hagenaars, R. Ranasinghe, F. Baart, G. Donchyts, and S. Aarninkhof. 2018. “The state of the world’s beaches.” Sci. Rep. 8 (1): 6641. https://doi.org/10.1038/s41598-018-24630-6.
Mariotti, G., and J. Carr. 2014. “Dual role of salt marsh retreat: Long-term and short-term resilience.” Water Resour. Res. 50 (4): 2963–2974. https://doi.org/10.1002/2013WR014676.
Mariotti, G., and S. Fagherazzi. 2010. “A numerical model for the coupled long-term evolution of salt marshes and tidal flats.” J. Geophys. Res. 115 (F1): F01004. https://doi.org/10.1029/2009JF001326.
Masetti, R., S. Fagherazzi, and A. Montanari. 2008. “Application of a barrier island translation model to the millennial-scale evolution of Sand Key, Florida.” Cont. Shelf Res. 28 (9): 1116–1126. https://doi.org/10.1016/j.csr.2008.02.021.
McBride, R. A., et al. 2013. “Morphodynamics of barrier systems: A synthesis.” In Vol. 10 of Treatise on geomorphology, 166–244. San Diego: Academic Press.
McCall, R. T., J. S. Van Thiel de Vries, N. G. Plant, A. R. Van Dongeren, J. A. Roelvink, D. M. Thompson, and A. J. Reniers. 2010. “Two-dimensional time dependent hurricane overwash and erosion modeling at Santa Rosa Island.” Coastal Eng. 57 (7): 668–683. https://doi.org/10.1016/j.coastaleng.2010.02.006.
McCann, S. 1972. “Reconnaissance survey of Hog Island, Prince Edward Island.” Marit. Sediments 8 (3): 107–113.
McCann, S. 1979. “Barrier Islands in the Southern Gulf of St. Lawrence, Canada.” In Barrier Islands: From the Gulf of St. Lawrence to the Gulf of Mexico, edited by S. P. Leatherman. Cambridge, MA: Academic Press.
McCarroll, R. J., G. Masselink, N. G. Valiente, T. Scott, M. Wiggins, J. A. Kirby, and M. A. Davidson. 2021. “A rules-based shoreface translation and sediment budgeting tool for estimating coastal change: ShoreTrans.” Mar. Geol. 435: 106466. https://doi.org/10.1016/j.margeo.2021.106466.
McNamara, D. E., and A. Keeler. 2013. “A coupled physical and economic model of the response of coastal real estate to climate risk.” Nat. Clim. Change 3 (6): 559–562. https://doi.org/10.1038/nclimate1826.
McNamara, D. E., and E. D. Lazarus. 2018. “Barrier Islands as coupled human-lanscape systems.” In Barrier dynamics and response to changing climate, edited by L. J. Moore and A. B. Murray, 363–383. New York: Springer.
McNamara, D. E., and B. T. Werner. 2008. “Coupled barrier island-resort model: 1. Emergent instabilities induced by strong human-landscape interactions.” J. Geophys. Res.: Earth Surf. 113 (1): 1–10.
Miller, J. K., and R. G. Dean. 2004. “A simple new shoreline change model.” Coastal Eng. 51 (7): 531–556. https://doi.org/10.1016/j.coastaleng.2004.05.006.
Montaño, J., et al. 2020. “Blind testing of shoreline evolution models.” Sci. Rep. 10 (1): 1–10. https://doi.org/10.1038/s41598-019-56847-4.
Moody, D. 1964. “Coastal morphology and processes in relation to the development of submarine sand ridges off Bethany Beach, Delaware.” Ph.D. thesis, Johns Hopkins Univ.
Moore, L. J., J. H. List, S. J. Williams, and D. Stolper. 2007. “Modeling Barrier Island response to sea-level rise in the outer banks, North Carolina.” In Proc., ASCE Coastal Sediments, 1153–1164. Reston, VA: ASCE.
Moore, L. J., J. H. List, S. J. Williams, and D. Stolper. 2010. “Complexities in barrier island response to sea level rise: Insights from numerical model experiments, North Carolina Outer Banks.” J. Geophys. Res. 115 (F2): F03004. https://doi.org/10.1029/2009JF001307.
Moore, L. J., and A. B. Murray. 2018. Barrier dynamics and response to changing climate. Cham, Switzerland: Springer International Publishing.
Moslow, T. F., and S. D., Jr. Heron. 1979. “Quaternary evolution of core banks, North Carolina: Cape lookout to new drum inlet.” In Barrier Islands: From the Gulf of St. Lawrence to the Gulf of Mexico, edited by S. P. Leatherman. Cambridge, MA: Academic Press.
Mull, J., and P. Ruggiero. 2014. “Estimating storm-induced dune erosion and overtopping along U.S. West Coast Beaches.” J. Coastal Res. 298 (6): 1173–1187. https://doi.org/10.2112/JCOASTRES-D-13-00178.1.
Murray, A. B. 2003. “Contrasting the goals, strategies, and predictions associated with simplified numerical models and detailed simulations.” In Prediction in geomorphology, edited by P. R. Wilcock and R. M. Iverson. Washington, DC: American Geophysical Union.
NASEM (National Academies of Sciences, Engineering, and Medicine). 2018. Understanding the long-term evolution of the coupled natural-human coastal system. Washington, DC: National Academies Press.
Nichols, R., and A. Marston. 1939. “Shoreline changes in Rhode Island produced by hurricane of September 21, 1938.” Geol. Soc. Am. Bull. 50 (9): 1357–1370. https://doi.org/10.1130/GSAB-50-1357.
Niedoroda, A. W., C. W. Reed, D. J. Swift, H. Arato, and K. Hoyanagi. 1995. “Modeling shore-normal large-scale coastal evolution.” Mar. Geol. 126 (1–4): 181–199. https://doi.org/10.1016/0025-3227(95)98961-7.
Nielsen, P. 2009. Coastal and estuarine processes. Singapore: World Scientific Publishing Company.
Nienhuis, J. H., L. G. Heijkers, and G. Ruessink. 2021. “Barrier breaching versus overwash deposition: Predicting the morphologic impact of storms on coastal barriers.” J. Geophys. Res.: Earth Surf. 126 (6): 1–17.
Nienhuis, J. H., and J. Lorenzo-Trueba. 2019. “Simulating barrier island response to sea level rise with the barrier island and inlet environment (BRIE) model V1.0.” Geosci. Model Dev. 12 (9): 4013–4030. https://doi.org/10.5194/gmd-12-4013-2019.
Nishi, R., and N. Kraus. 1996. “Mechanism and calculation of sand dune erosion by storms.” In Proc., 26th Int. Conf. on Coastal Engineering, 3034–3047. Reston, VA: ASCE.
Oertel, G. F. 1979. “Barrier Island development during the holocene recession, Southeastern United States.” In Barrier Islands: From the Gulf of St. Lawrence to the Gulf of Mexico, edited by S. P. Leatherman. Cambridge, MA: Academic Press.
Oppenheimer, M., et al. 2019. “Sea level rise and implications for low lying islands, coasts and communities coordinating.” In IPCC SR ocean and cyrosphere, edited by H.-O. Portner, D. Roberts, V. Masson-Delmotte, P. Zhai, M. Tignor, E. Poloczanska, K. Mintenbeck, A. Alegria, M. Nicolai, A. Okem, J. Petzold, B. Rama, and N. Weye. Cambridge, UK: Cambridge University Press.
Otvos, E., Jr. 1970. “Development and migration of barrier islands, Northern Gulf of Mexico.” Geol. Soc. Amer. Bull. 81 (1): 241–246. https://doi.org/10.1130/0016-7606(1970)81[241:DAMOBI]2.0.CO;2.
Over, J.-S., J. Brown, C. Sherwood, C. Hegermiller, P. Wernette, A. Ritchie, and J. Warrick. 2021. “A survey of storm-induced seaward-transport features observed during the 2019 and 2020 hurricane seasons.” Shore Beach 89 (2): 31–40. https://doi.org/10.34237/01.
Overton, M. F., J. S. Fisher, and M. A. Young. 1988. “Laboratory Investigation of Dune Erosion.” J. Waterway, Port, Coastal, Ocean Eng. 114 (3): 367–373. https://doi.org/10.1061/(ASCE)0733-950X(1988)114:3(367).
Overton, M. F., W. A. Pratikto, J. C. Lu, and J. S. Fisher. 1994. “Laboratory investigation of dune erosion as a function of sand grain size and dune density.” Coastal Eng. 23 (1–2): 151–165. https://doi.org/10.1016/0378-3839(94)90020-5.
Palalane, J., and M. Larson. 2020. “A long-term coastal evolution model with longshore and cross-shore transport.” J. Coastal Res. 36 (2): 411–423. https://doi.org/10.2112/JCOASTRES-D-17-00020.1.
Palmsten, M. L., and R. A. Holman. 2012. “Laboratory investigation of dune erosion using stereo video.” Coastal Eng. 60 (1): 123–135. https://doi.org/10.1016/j.coastaleng.2011.09.003.
Passeri, D. L., J. W. Long, N. G. Plant, M. V. Bilskie, and S. C. Hagen. 2018. “The influence of bed friction variability due to land cover on storm-driven barrier island morphodynamics.” Coastal Eng. 132 (Nov): 82–94. https://doi.org/10.1016/j.coastaleng.2017.11.005.
Pelnard-Considere, R. 1956. “Essai de theorie de l’evolution des formes de rivage en plages de sable et de galets.” Journées de l'hydraulique 4 (1): 289–298.
Pender, D., and H. Karunarathna. 2013. “A statistical-process based approach for modelling beach profile variability.” Coastal Eng. 81: 19–29. https://doi.org/10.1016/j.coastaleng.2013.06.006.
Penland, S., and R. Boyd. 1981. “Shoreline changes on the Louisiana Barrier Coast.” In Proc., Oceans, 209–219. Boston: IEEE.
Perlin, M., and R. G. Dean. 1979. “Prediction of beach planforms with littoral controls.” Proc. Coastal Eng. Conf. 2 (1): 1818–1838.
Perlin, M., and R. G. Dean. 1985. “3-D model of bathymetric response to structures.” J. Waterway, Port, Coastal, Ocean Eng. 111 (2): 153–170. https://doi.org/10.1061/(ASCE)0733-950X(1985)111:2(153).
Pierce, J. 1969. “Sediment budget along a barrier island chain.” Sediment. Geol. 3 (1): 5–16. https://doi.org/10.1016/0037-0738(69)90012-8.
Pierce, J. 1970. “Tidal inlets and washover fans.” J. Geol. 78.
Pilkey, O. H., and T. W. Davis. 1987. “An analysis of coastal recession models: North Carolina Coast.” In Sea-level fluctuation and coastal evolution, D. Nummedal, O. H. Pilkey, and J. D. Howard, 59–68. Tulsa, OK: SEPM Society for Sedimentary Geology.
Pilkey, O. H., W. J. Neal, J. T. Kelley, and J. A. G. Cooper. 2011. The world’s beaches: A global guide to the science of the shoreline.Berkeley, CA: University of California Press.
Pilkey, O. H., R. S. Young, S. R. Riggs, A. W. Smith, and W. D. Pilkey. 1993. “The concept of shoreface profile of equilibrium: A critical review.” J. Coastal Res. 9 (1): 255–278.
Quartel, S., B. G. Ruessink, and A. Kroon. 2007. “Daily to seasonal cross-shore behaviour of quasi-persistent intertidal beach morphology.” Earth Surf. Processes Landforms 32 (9): 1293–1307. https://doi.org/10.1002/(ISSN)1096-9837.
Ranasinghe, R. 2020. “On the need for a new generation of coastal change models for the 21st century.” Sci. Rep. 10 (1): 1–6. https://doi.org/10.1038/s41598-020-58376-x.
Ranasinghe, R., D. Callaghan, and M. J. Stive. 2012a. “Estimating coastal recession due to sea level rise: Beyond the Bruun rule.” Clim. Change 110 (3–4): 561–574. https://doi.org/10.1007/s10584-011-0107-8.
Ranasinghe, R., R. Holman, M. De Schipper, T. Lippmann, J. Wehof, T. M. Duong, D. Roelvink, and M. Stive. 2012b. “Quantifying nearshore morphological recovery time scales using argus video imaging: Palm Beach, Sydney and Duck, North Carolina.” In Proc., Coastal Engineering Conf., 1–7. Rome, Italy: International Conference on Coastal Engineering (ICCE).
Rathje, E. M., et al. 2017. “DesignSafe: New cyberinfrastructure for natural hazards engineering.” Nat. Hazard. Rev. 18 (3): 06017001. https://doi.org/10.1061/(ASCE)NH.1527-6996.0000246.
Raubenheimer, B. 2020. “Development of a nearshore extreme events reconnaissance community.” In Coastal Engineering Proc. Rome, Italy: International Conference on Coastal Engineering (ICCE)
Reeve, D. E., H. Karunarathna, S. Pan, J. M. Horrillo-Caraballo, G. Rózyński, and R. Ranasinghe. 2016. “Data-driven and hybrid coastal morphological prediction methods for mesoscale forecasting.” Geomorphology 256: 49–67. https://doi.org/10.1016/j.geomorph.2015.10.016.
Reeves, I. R., L. J. Moore, A. B. Murray, K. A. Anarde, and E. B. Goldstein. 2021. “Dune dynamics drive discontinuous barrier retreat.” Geophys. Res. Lett. 48 (13): 1–11. https://doi.org/10.1029/2021GL092958.
Robinet, A., D. Idier, B. Castelle, and V. Marieu. 2018. “A reduced-complexity shoreline change model combining longshore and cross-shore processes: The LX-Shore model.” Environ. Modell. Software 109 (Aug): 1–16. https://doi.org/10.1016/j.envsoft.2018.08.010.
Roelvink, D., B. Huisman, A. Elghandour, M. Ghonim, and J. Reyns. 2020. “Efficient modeling of complex sandy coastal evolution at monthly to century time scales.” Front. Mar. Sci. 7 (July): 1–20.
Roelvink, D., A. Reniers, A. van Dongeren, J. van Thiel de Vries, R. McCall, and J. Lescinski. 2009. “Modelling storm impacts on beaches, dunes and barrier islands.” Coastal Eng. 56 (11–12): 1133–1152. https://doi.org/10.1016/j.coastaleng.2009.08.006.
Roelvink, J. A. 2006. “Coastal morphodynamic evolution techniques.” Coastal Eng. 53 (2–3): 277–287. https://doi.org/10.1016/j.coastaleng.2005.10.015.
Rogers, L. J., L. J. Moore, E. B. Goldstein, C. J. Hein, J. Lorenzo-trueba, and A. D. Ashton. 2015. “Anthropogenic controls on overwash deposition: Evidence and consequences.” J. Geophys. Res.: Earth Surf. 120 (12): 2609–2624. https://doi.org/10.1002/2015JF003634.
Rosati, J. D., R. G. Dean, N. C. Kraus, and G. W. Stone. 2006. “Morphologic evolution of subsiding barrier island systems.” In Proc., 30th Int. Conf. on Coastal Engineering, 3963–3975. Singapore: World Scientific Publishing Company.
Rosati, J. D., R. G. Dean, and G. W. Stone. 2010. “A cross-shore model of barrier island migration over a compressible substrate.” Mar. Geol. 271 (1–2): 1–16. https://doi.org/10.1016/j.margeo.2010.01.005.
Rosati, J. D., R. G. Dean, and T. L. Walton. 2013. “The modified Bruun rule extended for landward transport.” Mar. Geol. 340: 71–81. https://doi.org/10.1016/j.margeo.2013.04.018.
Rosati, J. D., and G. W. Stone. 2009. “Geomorphologic evolution of Barrier Islands along the Northern U.S. Gulf of Mexico and implications for engineering design in barrier restoration.” J. Coastal Res. 251: 8–22. https://doi.org/10.2112/07-0934.1.
Rosen, P. S. 1979. “Aeolian dynamics of a barrier island system.” In Barrier Islands: From the Gulf of St. Lawrence to the Gulf of Mexico, edited by S. P. Leatherman. Cambridge, MA: Academic Press.
Ruessink, B. G., Y. Kuriyama, A. J. Reniers, J. A. Roelvink, and D. J. R. Walstra. 2007. “Modeling cross-shore sandbar behavior on the timescale of weeks.” J. Geophys. Res.: Earth Surf. 112 (3): 1–15.
Ruggiero, P., M. Buijsman, G. M. Kaminsky, and G. Gelfenbaum. 2010. “Modeling the effects of wave climate and sediment supply variability on large-scale shoreline change.” Mar. Geol. 273 (1–4): 127–140. https://doi.org/10.1016/j.margeo.2010.02.008.
Safak, I., J. H. List, J. C. Warner, and W. C. Schwab. 2017. “Persistent shoreline shape induced from offshore geologic framework: Effects of shoreface connected ridges.” J. Geophys. Res.: Oceans 122 (11): 8721–8738. https://doi.org/10.1002/2017JC012808.
Safak, I., J. C. Warner, and J. H. List. 2016. “Barrier island breach evolution: Alongshore transport and bay-ocean pressure gradient interactions.” J. Geophys. Res.: Oceans 121 (12): 8720–8730. https://doi.org/10.1002/jgrc.v121.12.
Sallenger, A. H. 2000. “Storm impact scale for barrier islands.” J. Coastal Res. 16 (3): 890–895.
Sánchez-Arcilla, A., and J. A. Jiménez. 1994. “Breaching in a wave-dominated barrier spit: The trabucador bar (north-eastern Spanish coast).” Earth Surf. Processes Landforms 19 (6): 483–498. https://doi.org/10.1002/(ISSN)1096-9837.
Schwartz, M. L. 1967. “The Bruun theory of sea-level rise as a cause of shore erosion.” J. Geol. 75 (1): 76–92. https://doi.org/10.1086/627232.
Schwartz, M. L. 1973. “Barrier islands.” In Benchmark papers in geology. Stroudsburg, PA: Dowden, Hutchinson & Ross.
Senechal, N., B. Castelle, and K. R. Bryan. 2017. “Storm clustering and beach response.” Chap. 8 in Coastal storms: Processes and impacts, edited by P. Ciavola and G. Coco. Hoboken, NJ: John Wiley & Sons.
Shaler, N. 1895. Beaches and tidal marshes of the Atlantic Coast. Woodstock, GA: American Book Company.
Shaw, L., P. Helmholz, D. Belton, and N. Addy. 2019. “Comparison of UAV LiDAR and imagery for beach monitoring.” Int. Arch. Photogramm. Remote Sens. Spatial Inf. Sci. 42 (2/W13): 589–596.
Shephard, F. P. 1950. Beach cycles in Southern California. Rep. No. Washington, DC: US Army Corps of Engineers.
Sherwood, C. R., et al. 2022. “Modeling the morphodynamics of coastal responses to extreme events: What shape are we in?” Annu. Rev. Mar. Science 14 (1): 457–492. https://doi.org/10.1146/marine.2022.14.issue-1.
Sherwood, C. R., J. W. Long, P. J. Dickhudt, P. S. Dalyander, D. M. Thompson, and N. G. Plant. 2014. “Inundation of Barrier Islands during a Hurricane: Observed water-level gradients and modeled seaward sand transport.” J. Geophys. Res. Earth Surf. 119: 1498–1515. https://doi.org/10.1002/2013JF003069.
Shin, C. S. 1996. “A one-dimensional model for storm breaching of barrier islands.” Dept. of Civil Engineering, Old Dominion Univ.
Simmons, J. A., K. D. Splinter, M. D. Harley, and I. L. Turner. 2019. “Calibration data requirements for modelling subaerial beach storm erosion.” Coastal Eng. 152 (Nov): 103507. https://doi.org/10.1016/j.coastaleng.2019.103507.
Smallegan, S. M., and J. L. Irish. 2017. “Barrier island morphological change by bay-side storm surge.” J. Waterway, Port, Coastal, Ocean Eng. 143 (5): 04017025. https://doi.org/10.1061/(ASCE)WW.1943-5460.0000413.
Soulsby, R. L. 1997. Dynamics of marine sands. London: Thomas Telford.
Splinter, K. D., J. T. Carley, A. Golshani, and R. Tomlinson. 2014. “A relationship to describe the cumulative impact of storm clusters on beach erosion.” Coastal Eng. 83: 49–55. https://doi.org/10.1016/j.coastaleng.2013.10.001.
Steetzel, H. J. 1993. “Cross-shore transport during storm surges.” Ph.D. dissertation, Dept. of Civil Engineering and Geosciences, Technische Univ. Delft.
Steetzel, H. J., H. de Vroeg, L. C. van Rijn, and J. M. Stam. 1998. “Morphological modelling using a modified multi-layer approach.” In Vol. 2 Proc., Coastal Eng. Conf., 2368–2381. Reston, VA: ASCE.
Stive, M. J., and H. J. de Vriend. 1995. “Modelling shoreface profile evolution.” Mar. Geol. 126 (1–4): 235–248. https://doi.org/10.1016/0025-3227(95)00080-I.
Stive, M. J., H. J. De Vriend, P. J. Cowell, and A. W. Niedoroda. 1995. “Behaviour-oriented models of shoreface evolution.” In Proc., Int. Conf. on Coastal Dynamics, 998–1005. Reston, VA: ASCE.
Stive, M. J., D. J. Roelvink, and H. de Vriend. 1990. “Large-scale coastal evolution concept.” In Proc., 22nd Int. Conf. on Coastal Engineering, 1962–1974. Reston, VA: ASCE.
Stolper, D., J. H. List, and E. R. Thieler. 2005. “Simulating the evolution of coastal morphology and stratigraphy with a new morphological-behaviour model (GEOMBEST).” Mar. Geol. 218 (1–4): 17–36. https://doi.org/10.1016/j.margeo.2005.02.019.
Storms, J. E., G. Weltje, J. van Dijke, C. Geel, and S. Kroonenberg. 2002. “Process-response modeling of wave-dominated coastal systems: Simulating evolution and stratigraphy on geological timescales.” J. Sediment. Res. 72 (2): 226–239. https://doi.org/10.1306/052501720226.
Stutz, M. L., and O. H. Pilkey. 2011. “Open-ocean barrier islands: Global influence of climatic, oceanographic, and depositional settings.” J. Coastal Res. 272: 207–222. https://doi.org/10.2112/09-1190.1.
Swift, D. J. 1975. “Barrier-island genesis: Evidence from the Central Atlantic Shelf, Eastern USA.” Sediment. Geol. 14 (1): 1–43. https://doi.org/10.1016/0037-0738(75)90015-9.
Tanaka, H., Suntoyo, and T. Nagasawa. 2002. “Sediment intrusion into Gamo Lagoon by wave overtopping.” In Proc., Coastal Engineering 2002, 823–835. Singapore: World Scientific Publishing.
Terwindt, J., and J. Battjes. 1990. “Research on large-scale coastal behavior.” In Proc., 22nd Int. Conf. on Coastal Engineering, 1975–1983. Reston, VA: ASCE.
Thieler, E. R., O. H. Pilkey, R. S. Young, D. M. Bush, and F. Chai. 2000. “The use of mathematical models to predict beach behavior for U.S. coastal engineering: A critical review.” J. Coastal Res. 16 (1): 48–70.
Thomas, R. C., and A. E. Frey. 2013. Shoreline change modeling using one-line models: General model comparison and literature review. Rep. No. ERDC/CHL CHETN-II-55. Vicksburg, MS: US Army Engineer Research and Development Center.
Toimil, A., P. Camus, I. J. Losada, G. Le Cozannet, R. J. Nicholls, D. Idier, and A. Maspataud. 2020. “Climate change-driven coastal erosion modelling in temperate sandy beaches: Methods and uncertainty treatment.” Earth Sci. Rev. 202 (Jan): 103110. https://doi.org/10.1016/j.earscirev.2020.103110.
Toimil, A., I. J. Losada, P. Camus, and P. Díaz-Simal. 2017. “Managing coastal erosion under climate change at the regional scale.” Coastal Eng. 128 (Aug): 106–122. https://doi.org/10.1016/j.coastaleng.2017.08.004.
Troy, C. D., Y. T. Cheng, Y. C. Lin, and A. Habib. 2021. “Rapid lake Michigan shoreline changes revealed by UAV LiDAR surveys.” Coastal Eng. 170 (Mar): 104008. https://doi.org/10.1016/j.coastaleng.2021.104008.
Turner, I. L., M. D. Harley, R. Almar, and E. W. Bergsma. 2021. “Satellite optical imagery in coastal engineering.” Coastal Eng. 167: 103919. https://doi.org/10.1016/j.coastaleng.2021.103919.
Turner, I. L., M. D. Harley, A. D. Short, J. A. Simmons, M. A. Bracs, M. S. Phillips, and K. D. Splinter. 2016. “A multi-decade dataset of monthly beach profile surveys and inshore wave forcing at Narrabeen, Australia.” Sci. Data 3 (1): 1–13. https://doi.org/10.1038/sdata.2016.24.
USACE. 1984. Shore protection manual: Vol. I. Rep. No. Washington, DC: USACE.
Van Baaren, P. F. 2007. “Influence of the wave period in the dune erosion model DUROSTA.” M.Sc. thesis, Dept of Civil Engineering and Geosciences, Delft Univ. of Technology.
van der Lugt, M. A., E. Quataert, A. van Dongeren, M. van Ormondt, and C. R. Sherwood. 2019. “Morphodynamic modeling of the response of two barrier islands to Atlantic hurricane forcing.” Estuarine Coastal Shelf Sci. 229 (May): 106404. https://doi.org/10.1016/j.ecss.2019.106404.
van Dongeren, A., et al. 2009. “MICORE: Dune erosion and overwash model validation with data from nine European field sites.” In Proc., Coastal Dynamics 2009, 1–15. Singapore: World Scientific Publishing Company.
van Ormondt, M., T. R. Nelson, C. J. Hapke, and D. Roelvink. 2020. “Morphodynamic modelling of the wilderness breach, Fire Island, New York. Part I: Model set-up and validation.” Coastal Eng. 157 (Dec): 103621. https://doi.org/10.1016/j.coastaleng.2019.103621.
van Rijn, L. 1993. “Principles of sediment transport in rivers, estuaries and coastal seas.” In Principles of sediment transport in rivers, estuaries and coastal seas, 1–17. Amsterdam, The Netherlands: Aqua Publications.
Van Rijn, L. C. 1984. “Sediment transport: Bed load transport.” J. Hydraul. Eng. 110 (10): 1431–1456. https://doi.org/10.1061/(ASCE)0733-9429(1984)110:10(1431).
Vellinga, P. 1986. “Beach and dune erosion during storm surges.” Ph.D. Dissertation, Dept. of Civil Engineering and Geosciences, Delft Univ. of Technology.
Vemulakonda, S. R., N. W. Scheffner, J. A. Earickson, and L. W. Chou. 1988. Kings bay coastal processes numerical model. Technical Rep. 88-3. Vicksburg, MI: US Army Engineer Waterways Experiment Station.
VGIN (Virginia Geographic Information Network). 2021. “Virginia Base Mapping Program (VBMP) orthoimagery.” Accessed October 1, 2021. https://vgin.vdem.virginia.gov/pages/base-mapping.
Visser, P. J. 1998. “Breach erosion in sand-dikes.” In Proc., Coastal Engineering Conf., 3516–3528. Reston, VA: ASCE.
Visser, P. J. 2000. “A model for breach erosion in sand-dikes.” In Proc., 27th Int. Conf. on Coastal Engineering, 3829–3842. Reston, VA: ASCE.
Vitousek, S., and P. L. Barnard. 2015. “A nonlinear, implicit one-line model to predict long-term shoreline change.” In Proc., Coastal Sediments 2015, 1–14. Singapore: World Scientific Publishing Company.
Vitousek, S., P. L. Barnard, P. Limber, L. Erikson, and B. Cole. 2017. “A model integrating longshore and cross-shore processes for predicting long-term shoreline response to climate change.” J. Geophys. Res.: Earth Surf. 122 (4): 782–806. https://doi.org/10.1002/2016JF004065.
Vitousek, S., L. Cagigal, J. Monta no, and A. Rueda. 2021. “The application of ensemble wave forcing to quantify uncertainty of shoreline change predictions.” J. Geophys. Res.: Earth Surf. 126 (7): 1–43.
Vos, K., M. D. Harley, K. D. Splinter, J. A. Simmons, and I. L. Turner. 2019. “Sub-annual to multi-decadal shoreline variability from publicly available satellite imagery.” Coastal Eng. 150 (Apr): 160–174. https://doi.org/10.1016/j.coastaleng.2019.04.004.
Walters, D., L. J. Moore, O. D. Vinent, S. Fagherazzi, and G. Mariotti. 2014. “Interactions between barrier islands and backbarrier marshes affect island system response to sea level rise: Insights from a coupled model.” J. Geophys. Res.: Earth Surf. 119 (9): 2013–2031. https://doi.org/10.1002/2014JF003091.
Wamsley, T. V., M. A. Cialone, J. M. Smith, B. A. Ebersole, and A. S. Grzegorzewski. 2009. “Influence of landscape restoration and degradation on storm surge and waves in southern Louisiana.” Nat. Hazard. 51 (1): 207–224. https://doi.org/10.1007/s11069-009-9378-z.
Wamsley, T. V., and N. C. Kraus. 2005. Coastal barrier island breaching, Part 2: Mechanical breaching and breach closure. Rep. No. Erdc/Chl Chetn-Iv-65. Vicksburg, MS: US Army Engineer Research and Development Center.
Warner, J. C., B. Armstrong, R. He, and J. B. Zambon. 2010. “Development of a coupled ocean-atmosphere-wave-sediment transport (COAWST) modeling system.” Ocean Modell. 35 (3): 230–244. https://doi.org/10.1016/j.ocemod.2010.07.010.
Warner, J. C., M. Olabarrieta, C. R. Sherwood, C. Hegermiller, and T. S. Kalra. 2018. “Investigations of morphological changes during hurricane sandy using a coupled modeling system.” In Proc., AGU Fall Meeting Abstracts. Washington, DC: American Geophysical Union.
Wartman, J., et al. 2020. “Research needs, challenges, and strategic approaches for natural hazards and disaster reconnaissance.” Front. Built Environ. 6 (Nov): 1–17.
Williams, P. 1978. “Laboratory development of a predictive relationship for washover volume on barrier island coastlines.” Ph.D. thesis, Dept. of Civil Engineering, Univ. of Delaware.
Wise, R. A., J. Smith, and M. Larson. 1996. SBEACH: Report 4 - Cross-shore transport under random waves and model validation with SUPERTANK and field data. Technical Rep. Vicksburg, MI: US Army Engineer Waterways Experiment Station.
Wolinsky, M. A., and A. B. Murray. 2009. “A unifying framework for shoreline migration: 2. Application to wave-dominated coasts.” J. Geophys. Res.: Earth Surf. 114 (1): 1–13.
Yates, M. L., R. T. Guza, and W. C. O’Reilly. 2009. “Equilibrium shoreline response: Observations and modeling.” J. Geophys. Res.: Oceans 114 (9): 1–16.
Young, R., O. H. Pilkey, D. Bush, and E. Thieler. 1995. “A discussion of the generalized model for simulating shoreline change (GENESIS).” J. Coastal Res. 11 (3): 875–886.
Zhang, K., and S. Leatherman. 2011. “Barrier island population along the U.S. Atlantic and gulf coasts.” J. Coastal Res. 27 (2): 356. https://doi.org/10.2112/JCOASTRES-D-10-00126.1.
Zinnert, J. C., S. M. Via, B. P. Nettleton, P. A. Tuley, L. J. Moore, and J. A. Stallins. 2019. “Connectivity in coastal systems: Barrier island vegetation influences upland migration in a changing climate.” Global Change Biol. 25 (7): 2419–2430. https://doi.org/10.1111/gcb.2019.25.issue-7.
Information & Authors
Information
Published In
Copyright
This work is made available under the terms of the Creative Commons Attribution 4.0 International license, https://creativecommons.org/licenses/by/4.0/.
History
Received: Aug 20, 2021
Accepted: Jan 15, 2023
Published online: May 30, 2023
Published in print: Sep 1, 2023
Discussion open until: Oct 30, 2023
ASCE Technical Topics:
Authors
Metrics & Citations
Metrics
Citations
Download citation
If you have the appropriate software installed, you can download article citation data to the citation manager of your choice. Simply select your manager software from the list below and click Download.