Exploring the Potential of Peracetic Acid for Wastewater Disinfection: Insights from a Pilot-Scale Study
Publication: Journal of Environmental Engineering
Volume 150, Issue 1
Abstract
Wastewater disinfection is a key treatment for the protection of the environment and public health from pathogenic infections. Peracetic acid (PAA) is an organic peroxyacid with a high oxidation potential that can be an alternative to conventional disinfectants. The aim of this pilot-scale study was to assess six disinfection conditions using PAA as a disinfectant agent of wastewater. To this end, a 6-month study was performed, and six disinfection conditions were studied: of PAA with 5, 10, and 15 min of contact time; of PAA with 10 and 15 min of contact time; and of PAA with 10 min of contact time. After the disinfection, an increase in the 5-day biochemical oxygen demand () and the chemical oxygen demand (COD) was observed; however, this was not proportional to the PAA dose because the higher doses tested did not result in a higher increment. In addition, PAA resulted in a slight decrease in pH. Nevertheless, the PAA impact on pH was not dependent on the PAA dose or the contact time. The PAA disinfection performance showed that doses of 10 and achieved Escherichia coli log reduction higher than 2 logs, and even higher than 3 logs when were tested with 10 and 15 min of contact time. The results showed also that the contact time was not a limiting factor for the PAA performance, at least for the contact times used in this study. This pilot-scale study proved that PAA can be an alternative disinfectant for wastewater.
Introduction
Wastewater disinfection plays a crucial role in eradicating or deactivating pathogenic microorganisms like bacteria or viruses (Antonelli et al. 2006). This treatment is of utmost importance because it safeguards both the environment and public health against pathogenic infections (Blanco-Canella et al. 2022; da Costa et al. 2014). Furthermore, this process is indispensable for facilitating the reuse of wastewater, whether for irrigation purposes or for recharging groundwater (da Costa et al. 2014). The importance of the disinfection step is well identified by the current legal requirements, for example, in Europe by the Regulation (EU) 2020/741 of the European Parliament and Council of the European Union (2020) and in the United States by the Guidelines for Water Reuse, which also state that disinfection is necessary for reuse (USEPA 2012). The most common wastewater disinfection processes use chlorine-based disinfectants (e.g., free chlorine, hypochlorite, chlorine dioxide, and chloramines), ozone, and ultraviolet (UV) radiation (Domínguez Henao et al. 2018c; Manoli et al. 2019; Xue et al. 2023).
Chlorine-based disinfection is undoubtedly the most used worldwide process for disinfection (da Costa et al. 2014; Domínguez Henao et al. 2018c; Freitas et al. 2021; Luukkonen and Pehkonen 2017) due to its effectiveness and affordability (Freitas et al. 2021; Luna-Pabello et al. 2009). However, chlorine use imposes public health risks due to the formation of harmful disinfection by-products (DBPs) (Collivignarelli et al. 2017; da Costa et al. 2014; Domínguez Henao et al. 2018c; Freitas et al. 2021) such as trihalomethanes and haloacetic acids (Domínguez Henao et al. 2018c; Freitas et al. 2021; Luo et al. 2020). The use of chloramines also results in nitrogenous DBP, namely, trichloronitromethane, which is genotoxic and cytotoxic (Xue et al. 2023).
Due to the concerns about chlorine DBP, many alternative techniques for wastewater disinfection have been studied and applied, such as chlorine dioxide, ozone, and UV radiation (da Costa et al. 2014; Domínguez Henao et al. 2018a). Chlorine dioxide is an alternative with high efficiency, although it is linked to operational constraints, such as preparation difficulties and storage risks (Luo et al. 2020). Ozonation and UV radiation are two possible alternatives, but they require expensive equipment and long implementation time, and they are also very sensitive to the physicochemical characteristics of the effluent (e.g., concentration of suspended solids, organic matter, and turbidity) (Ao et al. 2021; Beber de Souza et al. 2015; Domínguez Henao et al. 2018c). In addition, these two processes do not guarantee a disinfection residual concentration, maintaining the risk of bacteria regrowth in drainage systems or in supply systems for wastewater reuse purposes (Balachandran et al. 2021). By the aforementioned, a different disinfectant is needed, and its implementation is urgent. Recent studies have highlighted peracetic acid (PAA) as a promising alternative to the conventional methods of wastewater disinfection (Bell et al. 2023; da Silva et al. 2020; Kim and Huang 2020).
Organic peroxyacids such as PAA, performic acid, and permaleic acid can be alternatives to the conventional disinfectants used in wastewater (Chhetri et al. 2014; Pironti et al. 2021). PAA has a higher oxidation potential (1.96 V) compared to chlorine (1.4 V) or even to chlorine dioxide (1.5 V) (Ao et al. 2021; da Silva et al. 2020; Kim and Huang 2020) and is effective in the removal/inactivation of bacteria (e.g., Escherichia coli, Enterococci, Pseudomonas spp., and Salmonella spp.) and viruses (e.g., Coxsackievirus B3, coliphages, Norwalk virus) (Cavallini et al. 2013; da Silva et al. 2020; Dunkin et al. 2017; Kibbee and Örmeci 2020). In the disinfection process, PAA breaks down into hydrogen peroxide (), oxygen, water, and acetic acid (Dias et al. 2023; Freitas et al. 2021; Kibbee and Örmeci 2020), producing less toxic DBP than chlorine (Deng et al. 2022; Dong et al. 2022; Macêdo et al. 2020). This disinfectant is therefore considered a safe and environmentally friendly option (Antonelli et al. 2009; Dong et al. 2022; Freitas et al. 2021). In addition to the aspects mentioned, the low dependence on pH (Ao et al. 2021), the easy implementation (Antonelli et al. 2009; Ao et al. 2021), and its stability, long lead time, low corrosivity, wide availability, and reasonable cost (Chen and Pavlostathis 2019; Dias et al. 2023) are other advantages of this disinfectant. Recent studies point out that PAA, aside from being a disinfecting agent, can also be used in advanced oxidation processes for the removal of micropollutants and compounds of emerging concern (CEC) present in treated effluents allowing a safer wastewater reuse (Deng et al. 2022; Du et al. 2022; Maurício et al. 2020a, b). However, some disadvantages are also pointed out, such as the increasing organic content in the effluent (Pironti et al. 2021).
Despite the mentioned advantages, the study of PAA as a disinfectant has predominantly been conducted at a laboratory scale, with few studies in real-scale installations (Amerian et al. 2019; Wagner et al. 2002). According to Freitas et al. (2021), PAA achieved more effective results in the removal of E. coli and total coliforms than chlorine. Such outcome was also corroborated by Koivunen and Heinonen-Tanski (2005). In Freitas et al. (2021), of PAA and 15 min of contact time (CT) was the best disinfection condition. Within the same range of values, the work of Cavallini et al. (2013) supports that disinfection with a PAA dose lower than does not result in significant changes in the effluent characteristics [e.g., pH and 5-day biochemical oxygen demand ()]. There are also reports with lower PAA concentrations that were successful in the disinfection process, namely, the work of Kibbee and Örmeci (2020). This study showed that of PAA resulted in the total inactivation of E. coli, which was in line with the study by Luukkonen et al. (2014) that used PAA doses between 1.5 and and contact times between 10 and 15 min, stating that they were sufficient to achieve bacterial removal. Scaling up the PAA application, Pileggi et al. (2022) used of PAA and 35 min of contact time in a pilot-scale study, achieving an E. coli log reduction of 2.6. In the study conducted by Pileggi et al. (2022), it was shown that the effectiveness of PAA in removing E. coli was comparable to hypochlorite. However, hypochlorite exhibited a superior capability in removing viruses when compared to PAA. The variability of PAA initial concentration used in the mentioned studies may be related to the variable wastewater characteristics as supported by Domínguez Henao et al. (2018a) and Amerian et al. (2019), which reported that high total suspended solids (TSS) content results in a higher PAA decay rate and lower disinfection efficiency. It is known that chemical disinfection efficacy is frequently ruled by the exposure to the disinfectant, i.e., the disinfectant dose, which is the time-dependent concentration of disinfectant integrated over time typically measured in (Gujer and Von Gunten 2003). Therefore, it could be said that if a disinfectant dose is constant during the disinfection process, a steady disinfection performance will be achieved.
Commonly, the standard parameter used in drinking water, but also in some wastewater disinfection studies, is the disinfectant concentration multiplied by CT. CT is used to assess disinfectant efficacy and can evaluate a microorganism’s susceptibility to deactivation caused by a specific agent (Zhang et al. 2022; Newhart et al. 2021). However, in wastewater, the statement of upholding a constant dose is not evident because of the enormous variations of the wastewater quality and also because of possible side reactions between the disinfectant and the substances that are present in the wastewater (Manoli et al. 2019). This variability could lead, on one hand, to inadequate disinfection efficiency due to an initial and nearly instantaneous demand for disinfectant. On the other hand, overdosing cases can occur, resulting in higher operational and environmental costs (Manoli et al. 2019). Therefore, in wastewater studies it is crucial to consider the concentration of disinfectant, specifically focusing on the active or residual disinfectant concentration. Even though CT could be used to assess the disinfection effectiveness, in the studies with PAA the CT displays considerable variability. As a result, there are still uncertainties concerning some disinfectants kinetics in wastewater, namely, with PAA (Freitas et al. 2021; Pileggi et al. 2022; Ragazzo et al. 2020).
Despite the existing variability and the aforementioned considerations, PAA is poised to emerge as a next-generation disinfectant. However, to ensure a secure and efficient transition between disinfection agents, this potential must be substantiated by further studies on its viability at a larger scale (da Silva et al. 2020; Freitas et al. 2021; Kim and Huang 2020). To attain this objective, it is crucial to conduct additional research that comprehensively establishes the effectiveness of PAA as a wastewater disinfectant under diverse conditions. In line with this objective, the primary aim of this pilot-scale study was to assess the disinfection efficacy of PAA within an actual wastewater treatment plant (WWTP). This involved subjecting PAA to the daily operational conditions of wastewater treatment, including variations in wastewater quality and WWTP performance.
Materials and Methods
Pilot-Scale Study
The pilot installation was settled in a conventional municipal WWTP that treats a flow higher than (more than 200,000 inhabitants equivalent). The wastewater treatment process comprises preliminary, primary, and secondary treatment stages (activated sludge processes with nitrogen removal). The pilot-scale installation (Fig. 1) was assembled after the secondary clarifiers and before the final discharge point. The pilot installation had a capacity tank and was equipped with a vertical mixer [TIMSA (Madrid, Spain), Model TA] with a 0.75-kW electric motor and vertical coaxial reducer (600-mm diameter and 100-rpm velocity) and fed with a submersible pump with variable velocity (20 to ) (Dias et al. 2023). The PAA [OxyPure BIO—a mixture of 15% PAA, 24% , and 16% acetic acid—Evonik Operations GmbH (Essen, Germany)] was dosed using a metering pump with a capacity [Grundfos (Bjerringbro, Denmark) DME12-6AR-PP/E/E-F-311F, 600 kPa, with a single-phase power supply at 220 V] (Dias et al. 2023).
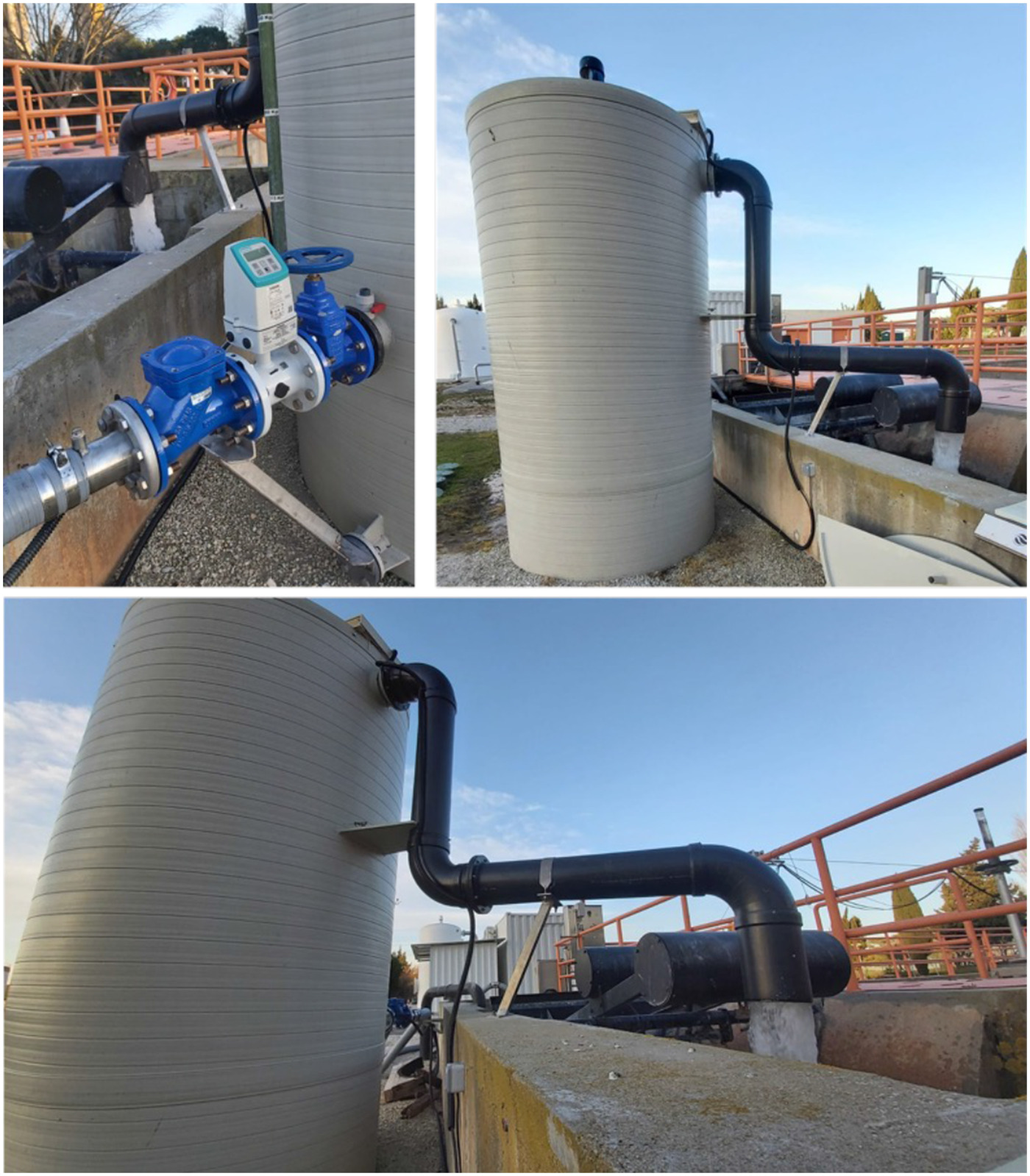
Study Conditions
The monitoring campaign was performed during 6 months to cover two different weather seasons. In this study, six disinfection conditions were tested (Table 1). Each condition was tested for 5 days (three samples per day) in which the wastewater quality parameters and the PAA residual concentration were determined.
Condition | PAA concentration () | Contact time (min) |
---|---|---|
D15T15 | 15 | 15 |
D15T10 | 15 | 10 |
D10T10 | 10 | 10 |
D10T15 | 10 | 15 |
D5T10 | 5 | 10 |
D15T5 | 15 | 5 |
Note: D = PAA concentration; and T = contact time.
Characterization of the Wastewater and PAA Residual Concentration
Before the disinfection with PAA, the following parameters were analyzed: , chemical oxygen demand (COD), TSS, ammonia, temperature, pH, turbidity, and E. coli. After the disinfection, all the mentioned parameters were also analyzed (except the ammonia content), and the PAA residual concentration was determined. Fig. 2 shows the flow diagram of the pilot-scale installation and sampling plan that was performed.
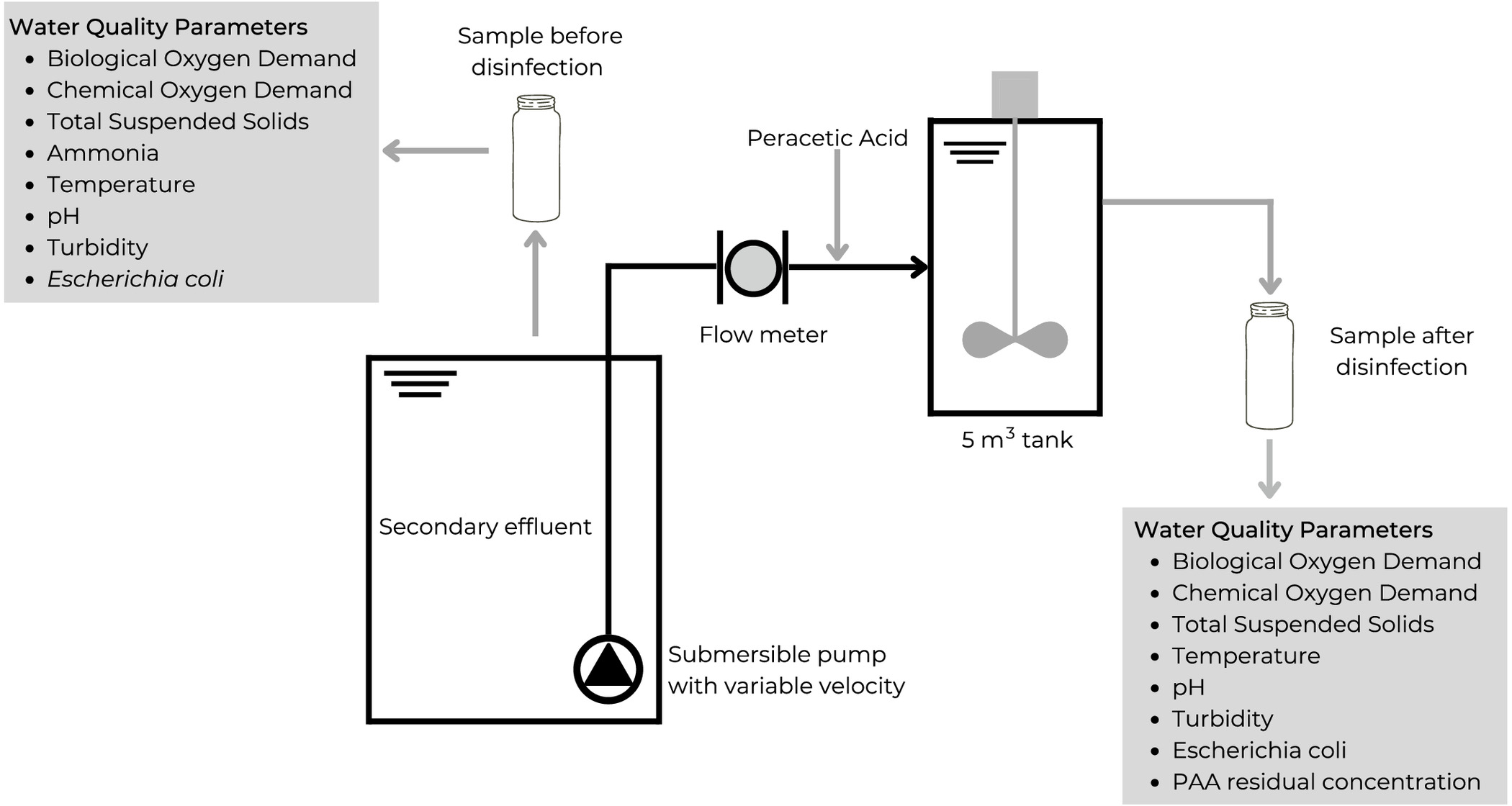
The and TSS were determined using the methods described in American Public Health Association (1999). COD and E. coli were determined according to the methods described by the ISO 6060:1989 (ISO 1989) and ISO 9308-2:2012 (ISO 2012), respectively. The ammonia was determined through molecular absorption spectrophotometry using the phenate method. Temperature and pH were determined by the potentiometric method, using a pH meter WTW (Weilheim, Germany) inoLab pH/ION 735 coupled with a temperature probe. The turbidity of the samples was measured according to the nephelometric method, using a portable turbidimeter 0-1000 NTU HANNA (Póvoa de Varzim, Portugal) Fast Tracker HI98703.
PAA residual concentration was determined according to the colorimetric method, adapted from the USEPA (1978) N,N-diethyl-p-phenylene diamine (DPD) method commonly used for chlorine determination (Method 330.5), as described by Domínguez-Henao et al. (2018d), in the spectrophotometer Merck (Darmstadt, Germany) Spectroquant Prove 100 at 530 nm. Based on this method, two calibration curves were obtained as shown in Table 2. These were applied according to the wavelength range, absorbance between 0.000 to 0.620 nm and 0.620 to 2.053 nm, respectively.
Calibration curve | Predicted | LOD () | LOQ () | Absorbance range | |
---|---|---|---|---|---|
0.996 | 0.995 | 0.14 | 0.48 | 0.000 to 0.620 | |
0.996 | 0.995 | 0.54 | 1.79 | 0.620 to 2.053 |
Note: ABS = absorbance; LOD = limit of determination; and LOQ = limit of quatification.
The PAA performance as a disinfectant was assessed through the E. coli log reduction (LR) achieved. The E. coli LR were determined using Eq. (1), where is the E. coli content before disinfection (MPN/100 mL) and is the E. coli content after disinfection (MPN/100 mL)
(1)
The CT analysis was conducted by multiplying the residual concentration of each sample by the corresponding contact time of PAA.
Statistical Analysis
The statistical evaluation of the results was made using one-way ANOVA and the Tukey test in SPSS v20 software. Both ANOVA and Tukey were used to assess the existence of significant differences in means between the conditions. The significance level was set at 5%, .
Results and Discussion
To understand the PAA applicability in wastewater disinfection, it was necessary to study the wastewater physical-chemical characteristics before and after the disinfection process to assess the impact of the wastewater variability on the disinfection performance. When thinking on a wider application, in different wastewater treatment facilities, PAA must be robust enough to comply with different wastewater legal requirements and to overcome the inherent variability. Such knowledge can only be attained through several experimental studies in the most different conditions to fully understand the mechanism of action and all the possible interferences (Zhang et al. 2022).
The wastewater characterization results, before disinfection, for each condition are presented in Table 3. Through the statistical analysis, no significant differences were identified between conditions for the results of and turbidity (one-way ANOVA, ). Therefore, it is possible to assume that these parameters were stable throughout the study. On the other hand, the results for COD, TSS, ammonia, temperature, pH, and E. coli showed differences between the studied conditions (one-way ANOVA, ).
Parameter | Value | Tested condition | |||||
---|---|---|---|---|---|---|---|
D15T15 | D15T10 | D10T10 | D10T15 | D5T10 | D15T5 | ||
() | Mean | 6.0 | 6.0 | 6.9 | 5.7 | 6.0 | 6.0 |
SD | 0.0 | 0.0 | 1.8 | 2.0 | 0.0 | 0.0 | |
Max | 6.0 | 6.0 | 11.1 | 11.4 | 6.0 | 6.0 | |
Min | 6.0 | 6.0 | 6.0 | 3.0 | 6.0 | 6.0 | |
COD () | Mean | 42.4 | 31.2 | 30.0 | 30.7 | 30.8 | 29.3 |
SD | 12.2 | 4.0 | 3.2 | 4.8 | 2.8 | 1.6 | |
Max | 66.0 | 40.4 | 35.4 | 39.0 | 40.9 | 30.8 | |
Min | 28.2 | 26.1 | 25.5 | 25.8 | 29.2 | 26.0 | |
TSS () | Mean | 7.2 | 4.6 | 4.7 | 4.4 | 4.4 | 3.8 |
SD | 1.3 | 1.3 | 1.2 | 1.9 | 2.6 | 1.5 | |
Max | 9.2 | 6.8 | 6.6 | 8.8 | 8.0 | 4.6 | |
Min | 5.0 | 2.6 | 2.8 | 1.4 | 0.0 | 1.2 | |
Ammonia () | Mean | 2.6 | 9.7 | 24.0 | 25.6 | 1.8 | 10.1 |
SD | 1.3 | 3.7 | 4.4 | 5.1 | 3.6 | 9.8 | |
Max | 6.2 | 13.0 | 30.0 | 32.0 | 9.2 | 25.0 | |
Min | 1.4 | 3.5 | 19.0 | 17.0 | 0.1 | 0.5 | |
Temperature (°C) | Mean | 21.4 | 23.2 | 25.5 | 24.8 | 25.6 | 26.5 |
SD | 1.0 | 1.8 | 0.6 | 0.4 | 1.1 | 0.5 | |
Max | 22.2 | 25.2 | 26.8 | 25.3 | 27.4 | 27.3 | |
Min | 19.2 | 20.2 | 24.7 | 24.1 | 23.5 | 26.0 | |
pH | Mean | 6.69 | 6.93 | 7.09 | 7.10 | 6.70 | 6.98 |
SD | 0.12 | 0.17 | 0.09 | 0.11 | 0.21 | 0.15 | |
Max | 6.97 | 7.23 | 7.24 | 7.38 | 7.00 | 7.20 | |
Min | 6.50 | 6.63 | 6.96 | 6.95 | 6.32 | 6.76 | |
Turbidity (NTU) | Mean | 1.6 | 1.4 | 1.2 | 1.2 | 1.4 | 1.2 |
SD | 0.6 | 0.4 | 0.2 | 0.3 | 0.6 | 0.2 | |
Max | 2.9 | 2.3 | 1.5 | 2.0 | 2.7 | 1.6 | |
Min | 0.9 | 0.9 | 0.9 | 0.8 | 0.6 | 0.9 | |
E. coli (MPN/100 mL) | Mean | 33,571 | 15,840 | 44,667 | 45,133 | 10,500 | 27,911 |
SD | 22,589 | 4,975 | 12,280 | 17,484 | 6,487 | 17,852 | |
Max | 73,000 | 25,000 | 65,000 | 77,000 | 24,000 | 58,000 | |
Min | 12,000 | 9,600 | 26,000 | 21,000 | 2,000 | 7,300 |
According to the Tukey test on COD results, only Condition D15T15 had significant differences from the others (Tukey, ), namely, with the highest mean value of COD. The same significant differences were identified in the TSS results (Tukey, ), where Condition D15T15 had the highest mean value of TSS. The statistical evaluation of the ammonia content showed that all the conditions had a significant difference with at least three of the studied conditions. In the pH statistical analysis, all the conditions had significant differences with at least two of the studied conditions.
Regarding wastewater temperature, Condition D15T15 also had significant differences when compared with the other studied conditions (Tukey, ). The same result was obtained for Condition D15T10 (Tukey, ). In these two conditions, the wastewater temperature was lower than the temperature of the other studied conditions.
Through the E. coli results it was possible to identify significant differences between Condition D15T10 and Conditions D15T15, D10T10, and D10T15 (Tukey, ). Condition D5T10 had significant differences from Conditions D15T15, D10T10, and D10T15 (Tukey, ). As can be demonstrated, Conditions D15T10 and D5T10 had an E. coli content lower than the other studied conditions. These results support the variability found in wastewater characteristics, even though all parameters are in accordance with the most recent legal European Union requirements for wastewater reuse, except for E. coli.
Among the effects of the use of PAA in the wastewater characteristics, the results showed an increase of the concentration in all conditions (Fig. 3), with significant differences between them (one-way ANOVA, ). According to the Tukey test results, Condition D5T10 had significant differences when compared with Conditions D10T10 and D15T5 (Tukey, ), while Condition D15T5 had significant differences with Condition D10T15 and D15T10 (Tukey, ). Condition D5T10 resulted in the lowest increase, , while Condition D15T5 resulted in the highest increase, . Such result is closely related to the PAA initial concentration, where higher doses resulted in higher increments, though such an increase was not proportional to the PAA dose because the increase verified in the conditions with of PAA was not significantly higher than the increase verified in the condition with .
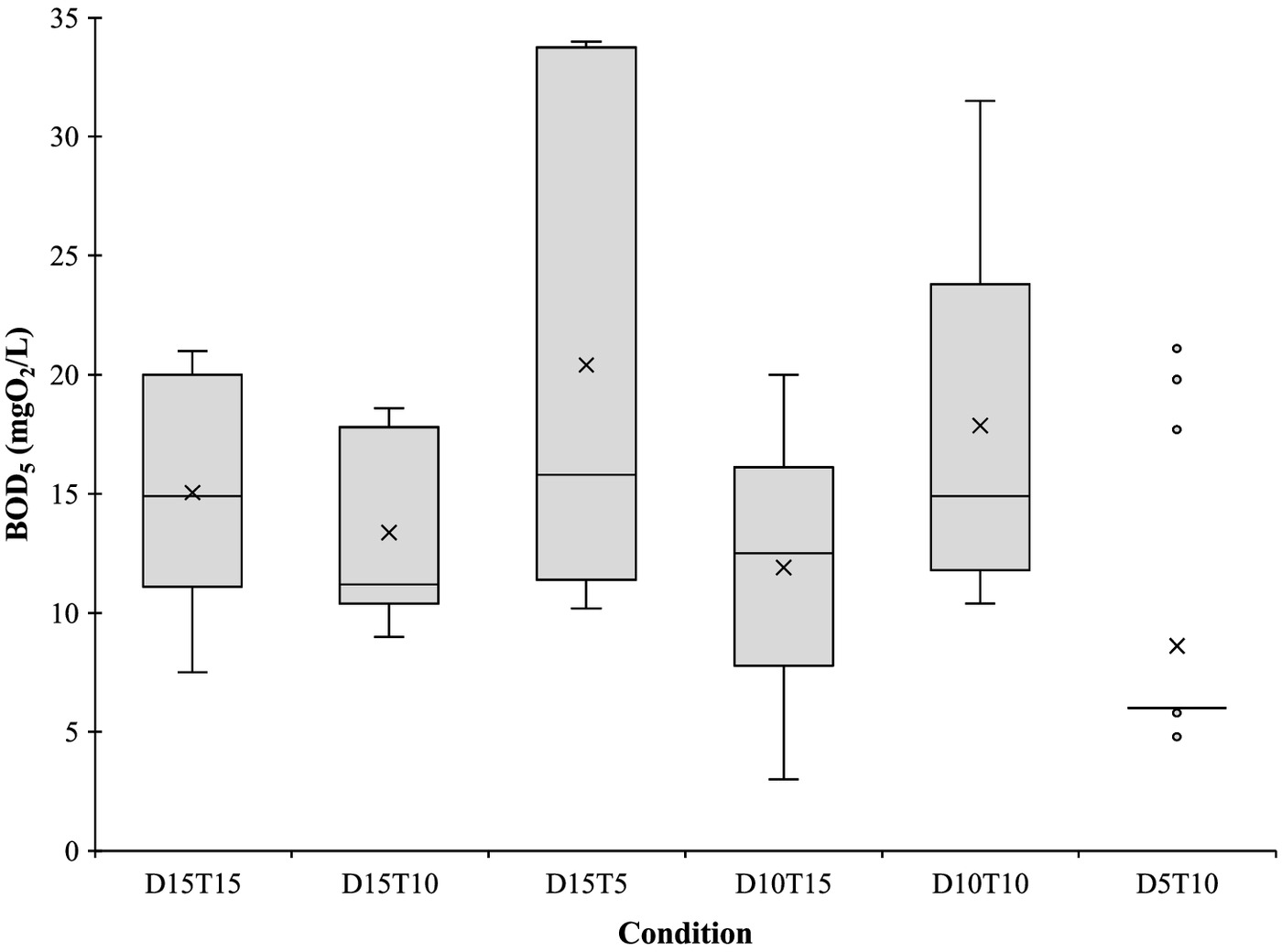
This trend is corroborated in the scientific literature where the increment of is explained by the presence of acetic acid, one of the PAA by-products, and also part of the commercial solution composition, which is a biodegradable compound (Luna-Pabello et al. 2009; Ragazzo et al. 2020; Wagner et al. 2002). On the other hand, oxygen is also released in PAA decomposition (secondary reaction of decomposition), which will increase the dissolved oxygen (DO) within the sample, affecting the estimation (even lowering the expected results) and thus underestimating DO oxygen consumption by bacteria (da Silva et al. 2020). Theoretically, the simple addition of of PAA (15% by weight) would increase of ; however, when combining the DO generated effect, the expected contribution decreases to of (VigorOX 2016). Such increment is reported in this study (Table 4), though the mean values were lower than the theoretical values, except for Condition D10T10. Due to this variability after PAA disinfection, this parameter is not recommended for the monitoring of biodegradable organic matter in wastewater (da Silva et al. 2020). However, for low PAA concentrations such as those used by Bell et al. (2023), the effect on BOD is apparently negligible.
Condition | Mean increase ( per mg PAA/L) |
---|---|
D15T15 | 0.60 |
D15T10 | 0.49 |
D10T10 | 1.10 |
D10T15 | 0.71 |
D5T10 | 0.52 |
D15T5 | 0.96 |
Similarly to the , variations on COD were expected because it is an organic matter–based parameter. Therefore, the addition of PAA also resulted in COD increment (Fig. 4), in all the studied conditions, with significant differences (one-way ANOVA, ). Condition D5T10 had significant differences when compared with the other studied conditions (Tukey, ), and had the lowest COD increase (), while the other five conditions resulted in a mean COD increase between 23.3 and .
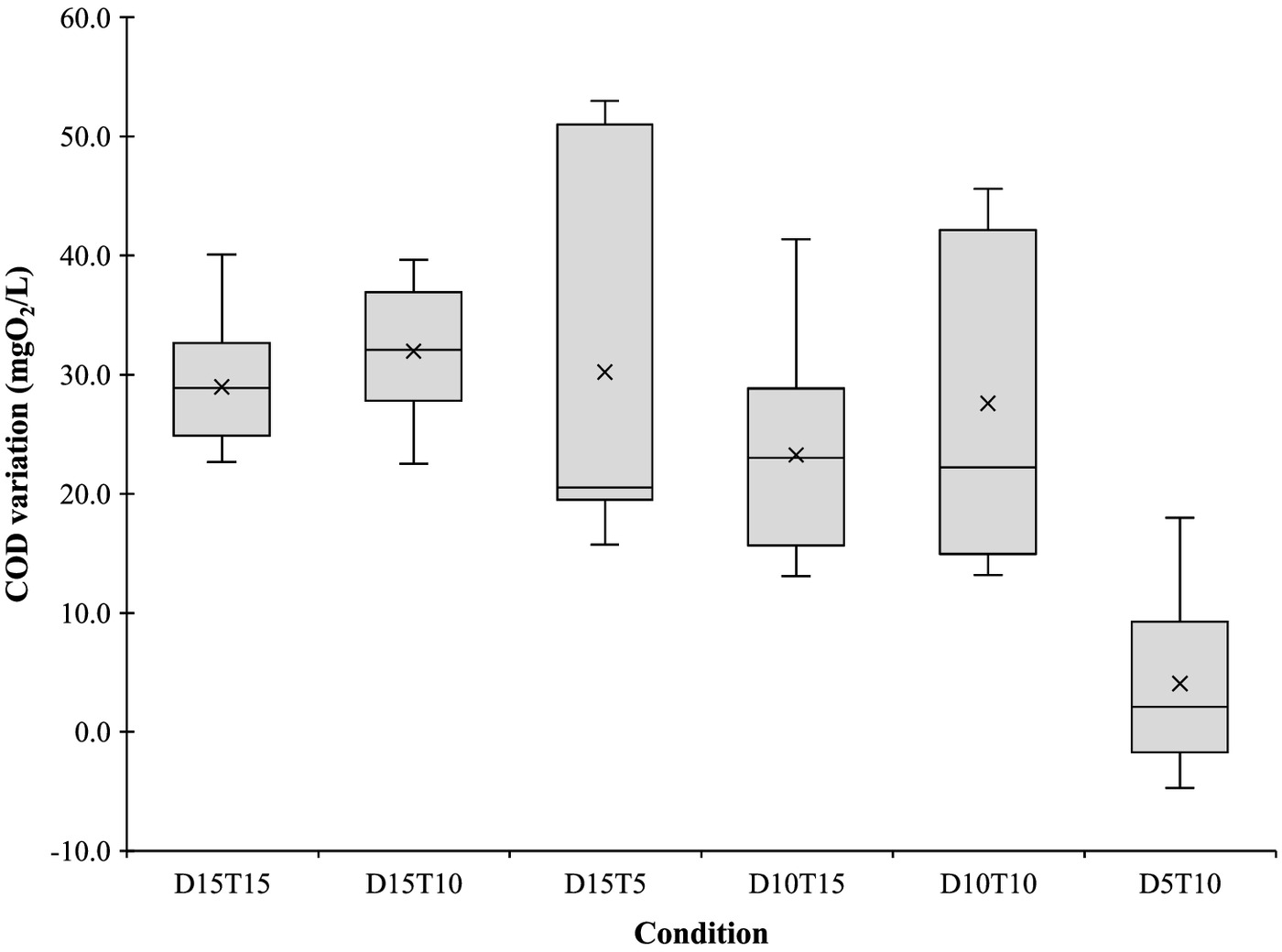
This effect on COD can also be explained by the presence of acetic acid and as DBP but also as part of the commercial solution composition. Theoretically, 1 mg of PAA represents a COD increment of 1.07 mg (Leite et al. 2021); however, Luukkonen et al. (2014), determined that PAA increases COD about . Such variability is dependent on the commercial solution composition, the ratio between PAA, acetic acid, and (Luukkonen et al. 2014), which supports the difference between this study and the reported works. For instance, Cavallini et al. (2013) referred an increment of for each of PAA applied. However, in the present study, the conditions with of PAA resulted in a higher COD increase, namely, in Condition D10T10 and in Condition D10T15. Wagner et al. (2002) reported that of PAA and 2 h of contact time resulted in a COD increment of , which was similar to the ones obtained, with the exception of Condition D5T10. Freitas et al. (2021) studied the use of of PAA with 15 min of contact time in an effluent treated in a hybrid system with different treatment zones (anaerobic, aerobic, and anoxic) and COD values increased . In the same study, using the same disinfection condition in an effluent treated in an upflow anaerobic sludge blanket, Freitas et al. (2021) reported a COD reduction of . The difference between the theoretical outcome results and the real ones may lie not only on the presence of acetic acid, but also the concentration (Leite et al. 2021). Leite et al. (2021) assessed the influence of in the COD results, and though theoretically COD value is 0.47 mg per mg of , no COD increment was experimentally detected until concentration reached . This theoretic underestimation can be related to the possible reactions between , and PAA with the dichromate ion (Leite et al. 2021). Such considerations must be addressed when using this parameter to monitor wastewater disinfection with PAA because commercial solutions contain three different oxidizing agents (PAA, , and acetic acid), with different concentrations, which may react differently with traditional COD tests (Leite et al. 2021).
Regarding the TSS content, there were significant differences between conditions before the disinfection, especially when the values of TSS were higher (one-way ANOVA, ). However, no significant differences were verified between the TSS values before and after in each condition (one-way ANOVA, ). Therefore, the outflow TSS conditions were similar to the inflow conditions, presented in the Table 2, concluding that PAA did not interfere with the TSS content. Similarly, in the turbidity results no significant differences were verified between conditions after the disinfection (one-way ANOVA, ), nor for the results before and after the disinfection for each condition (one-way ANOVA, ).
The disinfection with PAA resulted in a slight decrease of pH (Fig. 5), except in Condition D5T10, in which a slight pH increase was verified. The Tukey test performed revealed that the only condition that had significant differences from the other conditions was Condition D5T10 (Tukey, ). These results suggest that pH variation, due to PAA disinfection, was not dependent on the concentration or the contact time. For instance, Freitas et al. (2021) reported a pH decrease of 0.4 and 1.5 for a PAA concentration of and 15 min of contact time. Compared with the results of this study, pH variation was lower for the same condition (D15T15), with pH units between 0 and 0.2. Koivunen and Heinonen-Tanski (2005) obtained a pH decrease of 0.18 with of PAA in the tertiary effluent. This variation is close to the ones obtained in the present work in the conditions that resulted in the decrease of the pH. da Costa et al. (2014) reported a pH reduction of 0.13 when of PAA was added. Cavallini et al. (2013), Luna-Pabello et al. (2009), and Hey et al. (2012) also reported slight acidification of the effluent due to the addition of PAA. According to Cavallini et al. (2013), because PAA is a weak acid when combined with an alkaline effluent, the impact on pH is not significant, which may explain the low pH variation obtained in the present study. The pH decreases resulted from the presence of acetic acid formed during the PAA decomposition (da Silva et al. 2020; Hey et al. 2012; Luukkonen et al. 2014).
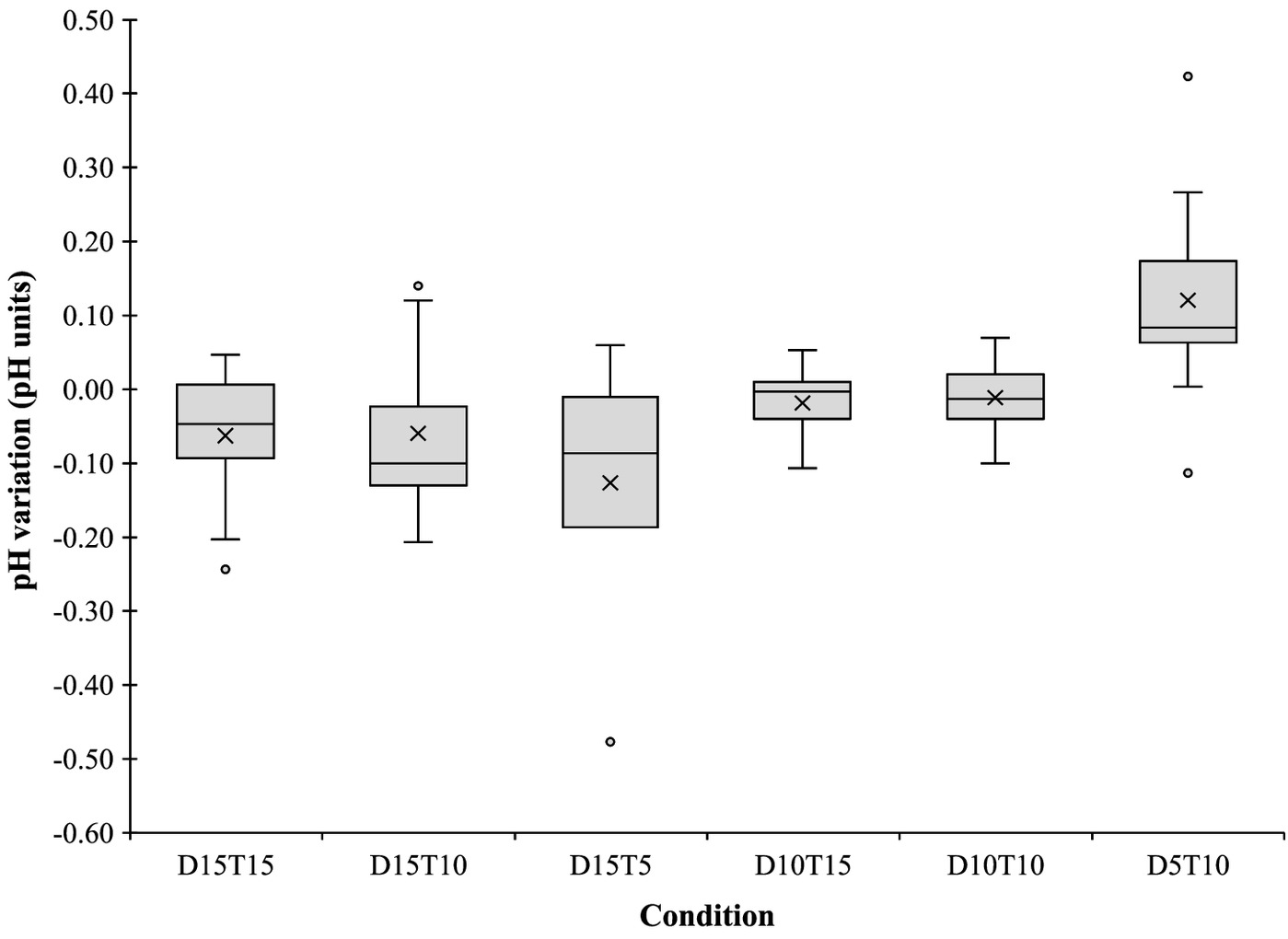
The E. coli LR obtained for each condition is shown in Fig. 6. As can be observed, there were differences between the conditions, supported by the ANOVA results (one-way ANOVA, ). The Tukey test showed that the LR obtained in Condition D10T10 was significantly different from LR obtained in Conditions D15T15, D15T10, and D5T10 (Tukey, ). For Condition D5T10, the results were significantly different from all the other conditions (Tukey, ). Within the disinfection performance, statistical results showed that no significant differences were found between the conditions with the same PAA dose, which may suggest that the contact time was not a limiting factor for the PAA performance, when 10 min minimum contact time is guaranteed. Also, the initial wastewater characteristics, previous to disinfection, did not seem to be reflected in the disinfection performance as expected and discussed in Section 3.1. Bonetta et al. (2017) studied the use of low PAA concentration, lower than , and 30 min of contact time, which achieved E. coli LR lower than 2 logs. Also, Ragazzo et al. (2020) reported E. coli LR of 1.6 for of PAA with 13 min of contact time. Those LRs were similar to the Condition D5T10 results, which may suggest that PAA doses lower than may not be efficient. On the other hand, according to Pileggi et al. (2022), with of PAA and 35 min of contact time, the inactivation of E. coli was 2.6 logs, and in this case, comparable with the results of Conditions D10T10, D10T15, and D15T5. As discussed in previous sections, wastewater characteristics may affect the PAA disinfection performance, and parameters such TSS or pH can be responsible for PAA inefficiency and variability. In the study performed by Freitas et al. (2021), using the D15T15 disinfection conditions in an effluent treated with a hybrid system with different treatment conditions (anaerobic, aerobic, and anoxic) obtained a LR of 3.9 logs, equivalent to the results of the present study. Using the same disinfection condition in an effluent treated with an upflow anaerobic sludge blanket the LR obtained by Freitas et al. (2021) was only 1.9 logs.
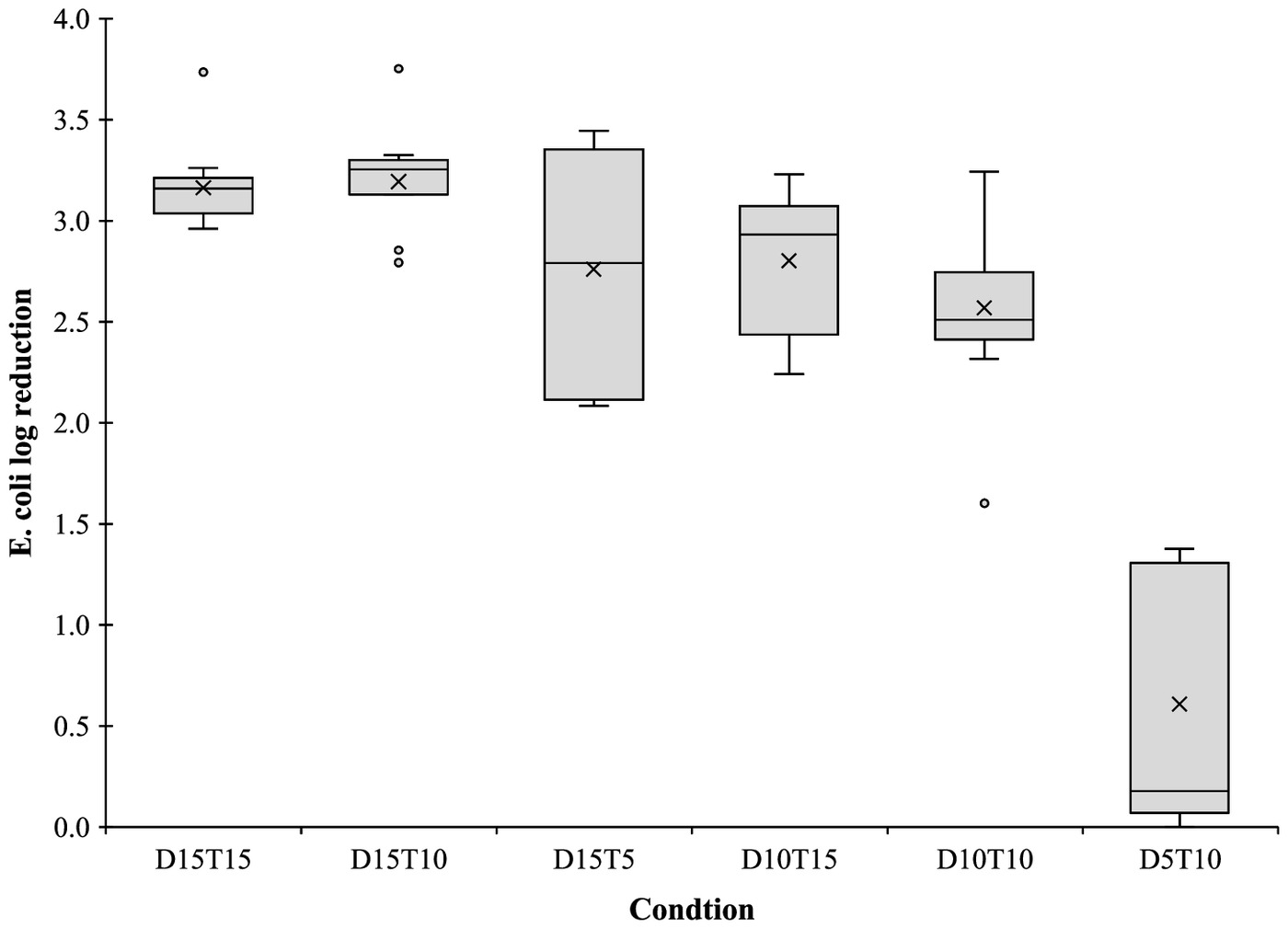
Within the disinfection performance evaluation, the PAA consumed was also assessed. According to Fig. 7, in all the studied conditions at least 40% of the added PAA was consumed (mean values). The ANOVA test performed for this consumption of PAA showed significant differences between the conditions (one-way ANOVA, ). According to the results of the Tukey test, the PAA consumed in Condition D15T10 was significantly different from Conditions D10T10, D5T10, and D15T5 (Tukey, ). This difference was expected because Condition D15T10 was the one with the lowest PAA consumption. The low PAA consumption in this condition may be explained by the low E. coli content verified before disinfection (Table 3). Condition D5T10 had the highest PAA consumption. In fact, in some of the samples the PAA residual concentration was lower than the limit of quantification (LOQ) (). Therefore, it was possible to conclude that even with a longer contact time, of PAA would not be enough to achieve higher LR of E. coli in this wastewater because almost the PAA was consumed.
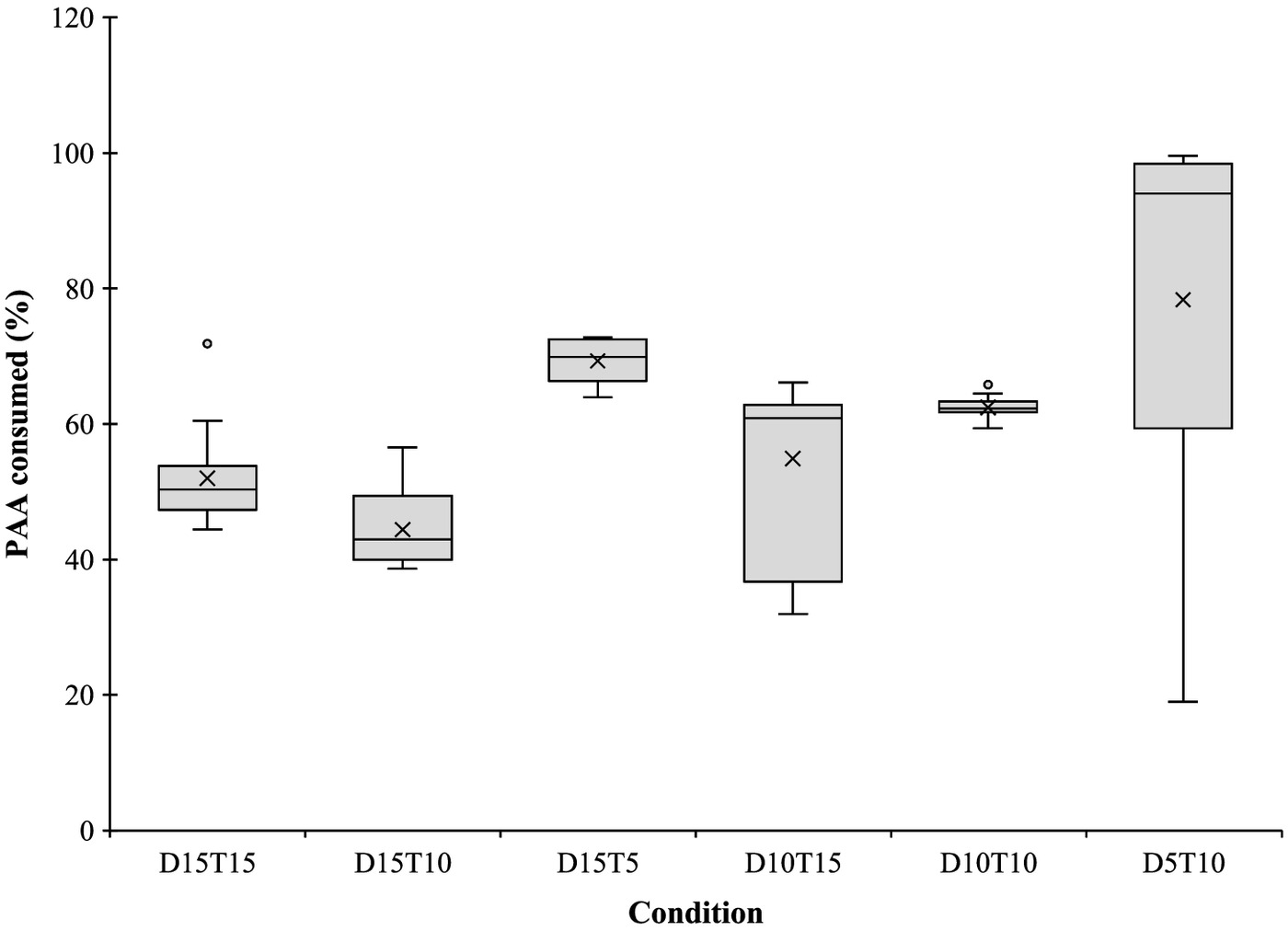
Corroborating this consumption behavior, Cavallini et al. (2013) reported consumption of 62%, 49%, 25%, and 27% for 5, 10, 15, and of PAA with 20 min of contact time, respectively. The main difference between Cavallini et al.’s (2013) results and those in the present study was the PAA consumed with doses of 15 and , which was lower. Also, da Costa et al. (2014) reported a PAA consumption of 83.6% using of PAA and 20 min of contact time. These results were similar to the results of Condition D5T10. On the other hand, Kibbee and Örmeci (2020), for PAA doses lower than the ones used in the present study, namely, 1.5 and , and 15 min of contact time, reported PAA consumption of 18% and 13%, respectively, below the expected, while Ragazzo et al. (2020) reported that independently of the PAA dose adopted, the consumption was not higher than 40%.
As referred to previously, wastewater characteristics may affect the PAA expected efficiency. Concerning turbidity and TSS content, it has been reported that a slight decrease in PAA disinfection efficiency is obtained when turbidity and TSS content increase significantly, which may be related to the well-known sheltering effect that suspended solids have on bacterial communities (Cavallini et al. 2013; Luukkonen et al. 2014). Although the most recent results, concerning the effect of turbidity in the PAA performance for E. coli inactivation, showed that at between 0.1 and 10 NTU the turbidity impact was not verified (Zhang et al. 2022). In the present work, the results of the PAA performance did not seem to be affected by the turbidity because the turbidity of the wastewater was always below 3 NTU (Table 3). As supported by Domínguez-Henao et al. (2018a) and Amerian et al. (2019), high TSS content results in a higher PAA decay rate and lower disinfection efficiency. This conclusion could be especially important for the TSS content because the presence of TSS, especially with small particle size, results in higher PAA consumption (Amerian et al. 2019). Domínguez-Henao et al. (2018a) tested the effect of of TSS in of PAA disinfection performance, obtaining a 0.33 log protection on E. coli. Although Condition D15T15 had a higher TSS content compared with the other conditions (Table 3), this difference did not lead to a higher consumption of PAA. Based on the low TSS content of the wastewater (Table 3), it was possible to assume that the sheltering effect was not expected to have a significant impact on the PAA performance. Further supporting these results is the research conducted by McFadden et al. (2017). In their study, a comparison between PAA and hypochlorite disinfection performance was carried out, revealing that PAA outperformed hypochlorite across all TSS size fractions. Notably, the disinfection efficiency of PAA demonstrated minimal susceptibility to variations, while the disinfection ability of hypochlorite was significantly compromised.
The wastewater pH can also interfere with the PAA stability and efficiency. The of PAA is 8.2; therefore, at acid to neutral pH solutions () this disinfectant is stable and more efficient. Indeed, based on the study conducted by Zhang et al. (2022), it was observed that the lowest pH tested, which was 6.5, exhibited a 4-log reduction within approximately 300 s of inactivation time. Interestingly, at a pH of 7.5, the performance remained quite comparable. However, above pH 7.5, Zhang et al. (2022) concluded that the PAA disinfection efficacy is compromised, which is also supported by findings in the work of McFadden et al. (2017). In the present work, the pH levels of the wastewater prior to disinfection, averaging between 6.5 and 7.5 (as shown in Table 3), further solidify this conclusion. Consequently, within this range of pH values, it can be confidently stated that the performance of PAA remained unaffected by the pH factor.
According to Chen and Pavlostathis (2019), temperature is also a parameter that may affect the PAA decay rate. The temperature increase could result in an increase of the PAA decay rate. This may explain some of the obtained results because the conditions in which lower PAA consumption occurred were the conditions with lower temperatures, namely, Conditions D15T15 and D15T10. Organic matter also could affect the PAA decay; however, not all organic compounds affect the PAA decay in the same way. For example, the proteins could influence the initial PAA demand (Domínguez Henao et al. 2018b). Because the wastewater before disinfection was considered the same in all the conditions, it was possible to assume that the organic content did not have a significant impact on the PAA consumption.
The Fig. 8 shows E. coli LR as a function of CT. It is possible to observe an increment in the E. coli LR with the increase of CT, followed by a stabilization phase. These values suggest that, beyond this threshold, higher CT might not yield proportional enhancements in disinfection efficacy. The obtained results are comparable with the work of Freitas et al. (2021), where an E. coli LR of 4 was achieved with a CT of in an effluent treated in a hybrid treatment system encompassing distinct treatment zones (anaerobic, aerobic, and anoxic). However, the results obtained did not show a relevant trend in the growth of E. coli LR, which indicates that such removals will not be achieved. In the same study, when the disinfection is carried out in an effluent treated within an upflow anaerobic sludge blanket configuration, despite using a higher CT value (), it failed to attain an E. coli LR of 2 (Freitas et al. 2021). This reveals the impact of the characteristics of the wastewater on the performance of the PAA. Koivunen and Heinonen-Tanski (2005) achieved E. coli LR of 2 with . This result is identical to the one obtained in the present work, in which it was possible to achieve this LR with approximately .
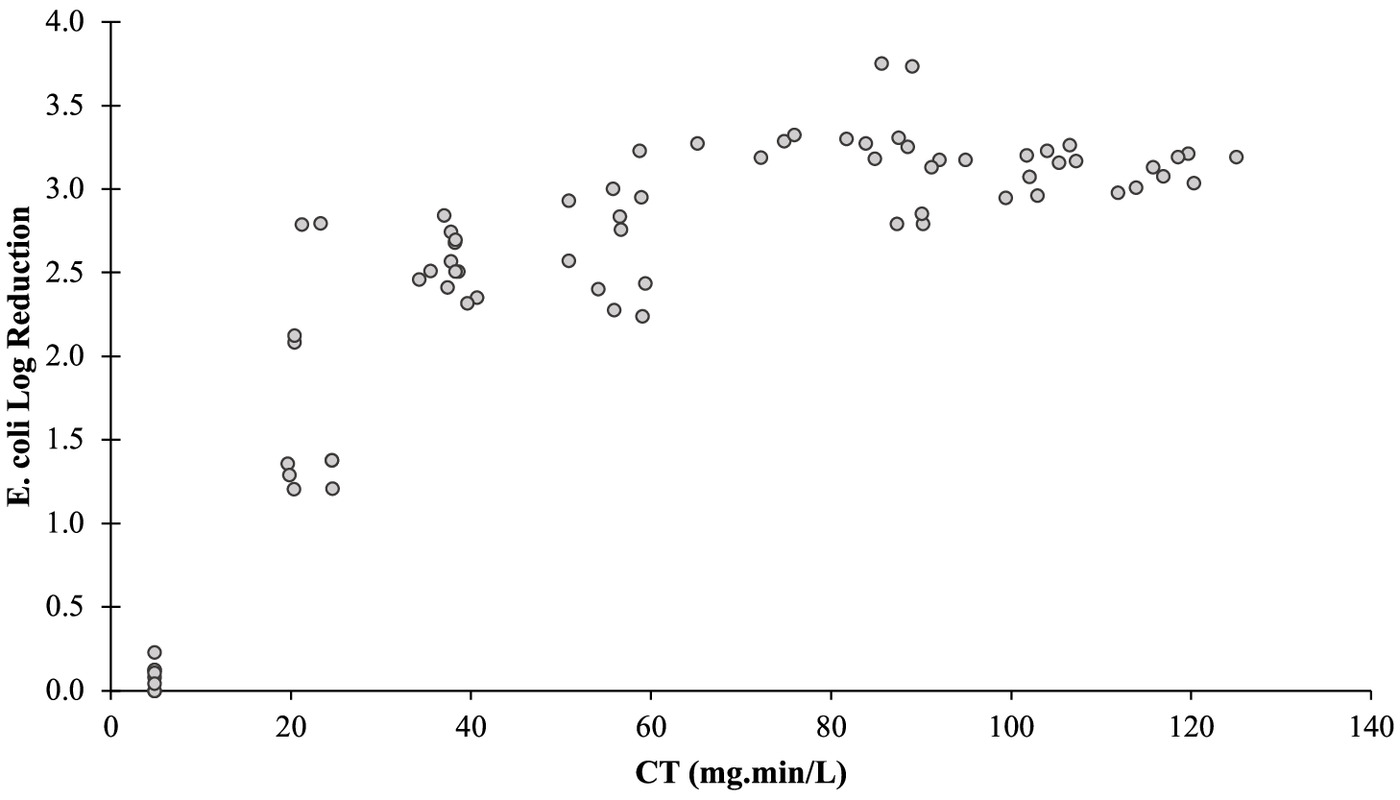
Although the analysis of CT in relation to E. coli LR serves as a prevalent method for characterizing disinfection efficiency, the outcomes exhibit a significant degree of variability. Consequently, lingering uncertainties persist regarding the PAA kinetics within wastewaters (Freitas et al. 2021; Pileggi et al. 2022; Ragazzo et al. 2020). Because CT depends on the residual concentration of disinfectant, it becomes essential to comprehend the specific characteristics of the wastewater that may have an impact on the consumption of PAA and its kinetics, as described by Newhart et al. (2021).
Conclusions
The use of PAA as a disinfectant resulted in the modification of wastewater quality. The addition of PAA resulted in an increase of the and the COD values, which was expected, and already reported. However, the obtained results showed that the increase was not proportional to the PAA concentration, a fact that may be influenced by the commercial PAA solution composition and due to the DBP interference. The results also showed that PAA addition resulted in a slight decrease in pH, also expected. Comparing the results between conditions, the PAA impact on pH was not dependent on the PAA concentrations or the tested contact times. The PAA disinfection performance showed that concentrations of 10 and achieved LR higher than 2 logs, and in some cases, higher than 3 logs, when was tested with 10 and 15 min of contact time. The analysis of the disinfection conditions showed that no significant differences were found between conditions with the same PAA dose. This fact may suggest that the contact time was not a determinant factor for the PAA performance when a minimum of 10 min is guaranteed. Such contact time flexibility could represent an important advantage for the full implementation of PAA in WWTPs. Consequently, further studies conducted in real WWTPs should prioritize the following aspects: (1) the influence of the physical-chemical properties of wastewater on PAA’s efficiency, (2) PAA’s efficacy in disinfecting other bacteria and viruses beyond E. coli, and (3) the potential toxicity associated with the use of this alternative disinfectant. By addressing these aspects, a more comprehensive understanding of PAA’s applicability and safety as a wastewater disinfectant can be obtained.
The results achieved with this pilot-scale study showed that PAA can be used as an alternative wastewater disinfectant and a promising disinfectant for wastewater reuse. This study supports that PAA is, in fact, an alternative for wastewater disinfection, even though long-term studies on full-scale installations will provide more information on PAA disinfection performance in different wastewater conditions, enhancing its potential.
Data Availability Statement
All data, models, or code that support the findings of this study are available from the corresponding author upon reasonable request.
Acknowledgments
The authors acknowledge and thank the support given to CENSE by the Portuguese Foundation for Science and Technology (FCT) through the strategic project UIDB/04085/2020 and through a Ph.D. grant by FCT for Rita Dias (SFRH/BD/148793/2019) and for Diogo Sousa (2022.11808.BD). The authors would like to thank Águas do Tejo Atlântico for the support.
References
Amerian, T., R. Farnood, S. Sarathy, and D. Santoro. 2019. “Effects of total suspended solids, particle size, and effluent temperature on the kinetics of peracetic acid decomposition in municipal wastewater.” Water Sci. Technol. 80 (12): 2299–2309. https://doi.org/10.2166/wst.2020.047.
American Public Health Association. 1999. “Standard methods for the examination of water and wastewater.” Public Health 51 (1): 940. https://doi.org/10.2105/AJPH.51.6.940-a.
Antonelli, M., V. Mezzanotte, and M. Panouillères. 2009. “Assessment of peracetic acid disinfected effluents by microbiotests.” Environ. Sci. Technol. 43 (17): 6579–6584. https://doi.org/10.1021/es900913t.
Antonelli, M., S. Rossi, V. Mezzanotte, and C. Nurizzo. 2006. “Secondary effluent disinfection: PAA long term efficiency.” Environ. Sci. Technol. 40 (15): 4771–4775. https://doi.org/10.1021/es060273f.
Ao, X., J. Eloranta, C.-H. Huang, D. Santoro, W. Sun, Z. Lu, and C. Li. 2021. “Peracetic acid-based advanced oxidation processes for decontamination and disinfection of water: A review.” Water Res. 188 (Jan): 116479. https://doi.org/10.1016/j.watres.2020.116479.
Balachandran, S., L. V. C. Charamba, K. Manoli, P. Karaolia, S. Caucci, and D. Fatta-Kassinos. 2021. “Simultaneous inactivation of multidrug-resistant Escherichia coli and enterococci by peracetic acid in urban wastewater: Exposure-based kinetics and comparison with chlorine.” Water Res. 202 (Sep): 117403. https://doi.org/10.1016/j.watres.2021.117403.
Beber de Souza, J., F. Queiroz Valdez, R. F. Jeranoski, C. M. D. S. Vidal, and G. S. Cavallini. 2015. “Water and wastewater disinfection with peracetic acid and uv radiation and using advanced oxidative process PAA/UV.” Int. J. Photoenergy 2015 (Aug): 1–7. https://doi.org/10.1155/2015/860845.
Bell, K. Y., A. B. Gruss, and D. Funk. 2023. “Peracetic acid: A tool for wastewater disinfection compliance.” J. Environ. Eng. 149 (7): 05023006. https://doi.org/10.1061/JOEEDU.EEENG-7212.
Blanco-Canella, P., G. Lama, M. A. Sanromán, and M. Pazos. 2022. “Disinfection through advance oxidation processes: Optimization and application on real wastewater matrices.” Toxics 10 (9): 512. https://doi.org/10.3390/toxics10090512.
Bonetta, S., C. Pignata, E. Lorenzi, M. De Ceglia, L. Meucci, S. Bonetta, G. Gilli, and E. Carraro. 2017. “Peracetic acid (PAA) disinfection: Inactivation of microbial indicators and pathogenic bacteria in a municipal wastewater plant.” Water 9 (6): 427. https://doi.org/10.3390/w9060427.
Cavallini, G. S., S. X. de Campos, J. B. de Souza, and C. M. de Sousa Vidal. 2013. “Evaluation of the physical–chemical characteristics of wastewater after disinfection with peracetic acid.” Water Air Soil Pollut. 224 (10): 1752. https://doi.org/10.1007/s11270-013-1752-5.
Chen, J., and S. G. Pavlostathis. 2019. “Peracetic acid fate and decomposition in poultry processing wastewater streams.” Bioresour. Technol. Rep. 7 (Feb): 100285. https://doi.org/10.1016/j.biteb.2019.100285.
Chhetri, R. K., D. Thornberg, J. Berner, R. Gramstad, U. Öjstedt, A. K. Sharma, and H. R. Andersen. 2014. “Chemical disinfection of combined sewer overflow waters using performic acid or peracetic acids.” Sci. Total Environ. 490 (Jun): 1065–1072. https://doi.org/10.1016/j.scitotenv.2014.05.079.
Collivignarelli, M. C., A. Abbà, I. Benigna, S. Sorlini, and V. Torretta. 2017. “Overview of the main disinfection processes for wastewater and drinking water treatment plants.” Sustainability 10 (2): 86. https://doi.org/10.3390/su10010086.
da Costa, J. B., S. Rodgher, L. A. Daniel, and E. L. G. Espíndola. 2014. “Toxicity on aquatic organisms exposed to secondary effluent disinfected with chlorine, peracetic acid, ozone and UV radiation.” Ecotoxicology 23 (9): 1803–1813. https://doi.org/10.1007/s10646-014-1346-z.
da Silva, W. P., T. D. Carlos, G. S. Cavallini, and D. H. Pereira. 2020. “Peracetic acid: Structural elucidation for applications in wastewater treatment.” Water Res. 168 (Jun): 115143. https://doi.org/10.1016/j.watres.2019.115143.
Deng, J., H. Wang, Y. Fu, and Y. Liu. 2022. “Phosphate-induced activation of peracetic acid for diclofenac degradation: Kinetics, influence factors and mechanism.” Chemosphere 287 (Mar): 132396. https://doi.org/10.1016/j.chemosphere.2021.132396.
Dias, R., D. Sousa, R. Lourinho, and R. Maurício. 2023. “Peracetic acid as a disinfectant for wastewater reuse—Regulation (EU) 2020/741 application on a pilot-scale.” Environ. Monit. Assess. 195 (6): 697. https://doi.org/10.1007/s10661-023-11313-7.
Domínguez Henao, L., M. Cascio, A. Turolla, and M. Antonelli. 2018a. “Effect of suspended solids on peracetic acid decay and bacterial inactivation kinetics: Experimental assessment and definition of predictive models.” Sci. Total Environ. 643 (Mar): 936–945. https://doi.org/10.1016/j.scitotenv.2018.06.219.
Domínguez Henao, L., R. Delli Compagni, A. Turolla, and M. Antonelli. 2018b. “Influence of inorganic and organic compounds on the decay of peracetic acid in wastewater disinfection.” Chem. Eng. J. 337 (Jul): 133–142. https://doi.org/10.1016/j.cej.2017.12.074.
Domínguez Henao, L., A. Turolla, and M. Antonelli. 2018c. “Disinfection by-products formation and ecotoxicological effects of effluents treated with peracetic acid: A review.” Chemosphere 213 (May): 25–40. https://doi.org/10.1016/j.chemosphere.2018.09.005.
Domínguez-Henao, L., A. Turolla, D. Monticelli, and M. Antonelli. 2018d. “Assessment of a colorimetric method for the measurement of low concentrations of peracetic acid and hydrogen peroxide in water.” Talanta 183 (Mar): 209–215. https://doi.org/10.1016/j.talanta.2018.02.078.
Dong, S., Y. Liu, L. Feng, and L. Zhang. 2022. “Oxidation of pyrazolone pharmaceuticals by peracetic acid: Kinetics, mechanism and genetic toxicity variations.” Chemosphere 291 (Aug): 132947. https://doi.org/10.1016/j.chemosphere.2021.132947.
Du, P., W. Liu, Z. Rao, and J. Wang. 2022. “Accelerated oxidation of organic micropollutants during peracetic acid treatment in the presence of bromide ions.” ACS ES&T Water 2 (2): 320–328. https://doi.org/10.1021/acsestwater.1c00355.
Dunkin, N., S. Weng, C. G. Coulter, J. G. Jacangelo, and K. J. Schwab. 2017. “Reduction of human norovirus GI, GII, and surrogates by peracetic acid and monochloramine in municipal secondary wastewater effluent.” Environ. Sci. Technol. 51 (20): 11918–11927. https://doi.org/10.1021/acs.est.7b02954.
European Parliament and Council of the European Union. 2020. Regulation (EU) 2020/741 of the European Parliament and of the Council of 25 May 2020 on minimum requirements for water reuse (Text with EEA relevance). Brussels, Belgium: Official Journal of the European Union.
Freitas, B. D. O., L. de Souza Leite, and L. A. Daniel. 2021. “Chlorine and peracetic acid in decentralized wastewater treatment: Disinfection, oxidation and odor control.” Process Saf. Environ. Prot. 146 (Jun): 620–628. https://doi.org/10.1016/j.psep.2020.11.047.
Gujer, W., and U. Von Gunten. 2003. “A stochastic model of an ozonation reactor.” Water Res. 37 (7): 1667–1677. https://doi.org/10.1016/S0043-1354(02)00456-6.
Hey, G., A. Ledin, J. L. C. Jansen, and H. R. Andersen. 2012. “Removal of pharmaceuticals in biologically treated wastewater by chlorine dioxide or peracetic acid.” Environ. Technol. 33 (9): 1041–1047. https://doi.org/10.1080/09593330.2011.606282.
ISO. 1989. Water quality—Determination of the chemical oxygen demand. ISO 6060:1989. Geneva: ISO.
ISO. 2012. Water quality—Enumeration of Escherichia coli and coliform bacteria—Part 2: Most probable number method. ISO 9308-2:2012. Geneva: ISO.
Kibbee, R., and B. Örmeci. 2020. “Peracetic acid (PAA) and low-pressure ultraviolet (LP-UV) inactivation of Coxsackievirus B3 (CVB3) in municipal wastewater individually and concurrently.” Water Res. 183 (May): 116048. https://doi.org/10.1016/j.watres.2020.116048.
Kim, J., and C.-H. Huang. 2020. “Reactivity of peracetic acid with organic compounds: A critical review.” ACS ES&T Water 1 (Jun): 15–33. https://doi.org/10.1021/acsestwater.0c00029.
Koivunen, J., and H. Heinonen-Tanski. 2005. “Peracetic acid (PAA) disinfection of primary, secondary and tertiary treated municipal wastewaters.” Water Res. 39 (18): 4445–4453. https://doi.org/10.1016/j.watres.2005.08.016.
Leite, L. D. S., M. D. Tango, J. A. Zanetoni Filho, M. T. Hoffmann, and L. A. Daniel. 2021. “Implications of COD analysis use in the peracetic acid-based wastewater treatment.” Water Sci. Technol. 84 (5): 1270–1279. https://doi.org/10.2166/wst.2021.300.
Luna-Pabello, V. M., M. M. Ríos, B. Jiménez, and M. T. Orta De Velasquez. 2009. “Effectiveness of the use of Ag, Cu and PAA to disinfect municipal wastewater.” Environ. Technol. 30 (2): 129–139. https://doi.org/10.1080/09593330802422506.
Luo, Y., L. Feng, Y. Liu, and L. Zhang. 2020. “Disinfection by-products formation and acute toxicity variation of hospital wastewater under different disinfection processes.” Sep. Purif. Technol. 238 (May): 116405. https://doi.org/10.1016/j.seppur.2019.116405.
Luukkonen, T., and S. O. Pehkonen. 2017. “Peracids in water treatment: A critical review.” Crit. Rev. Environ. Sci. Technol. 47 (1): 1–39. https://doi.org/10.1080/10643389.2016.1272343.
Luukkonen, T., J. Teeriniemi, H. Prokkola, J. Rämö, and U. Lassi. 2014. “Chemical aspects of peracetic acid based wastewater disinfection.” Water SA 40 (1): 73–80. https://doi.org/10.4314/wsa.v40i1.9.
Macêdo, L. P. R., A. S. P. Dornelas, M. M. Vieira, J. S. D. J. Ferreira, R. A. Sarmento, and G. S. Cavallini. 2020. “Effects of lethal and sublethal concentrations of peracetic acid and active chlorine of calcium hypochlorite on Chironomus xanthus.” Chemosphere 256 (Sep): 127171. https://doi.org/10.1016/j.chemosphere.2020.127171.
Manoli, K., S. Sarathy, R. Maffettone, and D. Santoro. 2019. “Detailed modeling and advanced control for chemical disinfection of secondary effluent wastewater by peracetic acid.” Water Res. 153 (Mar): 251–262. https://doi.org/10.1016/j.watres.2019.01.022.
Maurício, R., J. Jorge, R. Dias, J. P. P. Noronha, L. Amaral, M. A. A. Daam, A. P. Mano, and M. S. S. Diniz. 2020a. “The use of peracetic acid for estrogen removal from urban wastewaters: E2 as a case study.” Environ. Monit. Assess. 192 (2): 114. https://doi.org/10.1007/s10661-020-8079-7.
Maurício, R., F. Semedo, R. Dias, J. P. Noronha, L. Amaral, M. A. Daam, A. P. Mano, and M. S. Diniz. 2020b. “Efficacy assessment of peracetic acid in the removal of synthetic 17α-ethinyl estradiol contraceptive hormone in wastewater.” J. Environ. Sci. 89 (Apr): 1–8. https://doi.org/10.1016/j.jes.2019.09.019.
McFadden, M., J. Loconsole, A. J. Schockling, R. Nerenberg, and J. P. Pavissich. 2017. “Comparing peracetic acid and hypochlorite for disinfection of combined sewer overflows: Effects of suspended-solids and pH.” Sci. Total Environ. 599–600 (Jun): 533–539. https://doi.org/10.1016/j.scitotenv.2017.04.179.
Newhart, K. B., J. E. Goldman-Torres, D. E. Freedman, K. B. Wisdom, A. S. Hering, and T. Y. Cath. 2021. “Prediction of peracetic acid disinfection performance for secondary municipal wastewater treatment using artificial neural networks.” ACS ES&T Water 1 (2): 328–338. https://doi.org/10.1021/acsestwater.0c00095.
Pileggi, V., J. R. Bicudo, M. Nowierski, M. Manoharan, G. Lai, T. Fletcher, and A. Simhon. 2022. “Side-stream comparison of peracetic acid and chlorine as hypochlorite for disinfection of municipal wastewater effluent at a full-scale treatment facility, Ontario, Canada.” ACS ES&T Eng. 2 (8): 1465–1474. https://doi.org/10.1021/acsestengg.2c00005.
Pironti, C., F. Dell’Annunziata, R. Giugliano, V. Folliero, M. Galdiero, M. Ricciardi, O. Motta, A. Proto, and G. Franci. 2021. “Comparative analysis of peracetic acid (PAA) and permaleic acid (PMA) in disinfection processes.” Sci. Total Environ. 797 (Mar): 149206. https://doi.org/10.1016/j.scitotenv.2021.149206.
Ragazzo, P., N. Chiucchini, V. Piccolo, M. Spadolini, S. Carrer, F. Zanon, and R. Gehr. 2020. “Wastewater disinfection: Long-term laboratory and full-scale studies on performic acid in comparison with peracetic acid and chlorine.” Water Res. 184 (Oct): 116169. https://doi.org/10.1016/j.watres.2020.116169.
USEPA. 1978. EPA methods and guidance for analysis of water, version 2.0. Washington, DC: USEPA.
USEPA. 2012. “2012 guidelines for water reuse.” Accessed March 23, 2023. https://www.epa.gov/sites/default/files/2019-08/documents/2012-guidelines-water-reuse.pdf.
VigorOX. 2016. “The impact of peracetic acid on receiving waters: Biochemical oxygen demand and dissolved oxygen.” Disinfection Forum 11. Accessed April 14, 2023. https://www.peroxychem.com/media/164735/january-2016-paa-and-bod-and-do.pdf.
Wagner, M., D. Brumelis, and R. Gehr. 2002. “Disinfection of wastewater by hydrogen peroxide or peracetic acid: Development of procedures for measurement of residual disinfectant and application to a physicochemically treated municipal effluent.” Water Environ. Res. 74 (1): 33–50. https://doi.org/10.2175/106143002X139730.
Xue, B., X. Guo, J. Cao, S. Yang, Z. Qiu, J. Wang, and Z. Shen. 2023. “The occurrence, ecological risk, and control of disinfection by-products from intensified wastewater disinfection during the COVID-19 pandemic.” Sci. Total Environ. 900 (Aug): 165602. https://doi.org/10.1016/j.scitotenv.2023.165602.
Zhang, S., L. Jiang, H. Li, J. Zhang, T. Sun, Y. Dong, and N. Ding. 2022. “Disinfection kinetics of peracetic acid inactivation of pathogenic bacteria in water.” Water Cycle 3 (Dec): 79–85. https://doi.org/10.1016/j.watcyc.2022.05.002.
Information & Authors
Information
Published In
Copyright
This work is made available under the terms of the Creative Commons Attribution 4.0 International license, https://creativecommons.org/licenses/by/4.0/.
History
Received: May 23, 2023
Accepted: Sep 7, 2023
Published online: Nov 6, 2023
Published in print: Jan 1, 2024
Discussion open until: Apr 6, 2024
ASCE Technical Topics:
- Acids
- Business management
- Chemical compounds
- Chemical processes
- Chemical properties
- Chemicals
- Chemistry
- Disinfection
- Environmental engineering
- Medical wastes
- Oxygen demand
- pH
- Pollutants
- Practice and Profession
- Public administration
- Public health and safety
- Solid wastes
- Wastes
- Wastewater management
- Wastewater treatment
- Water treatment
Authors
Metrics & Citations
Metrics
Citations
Download citation
If you have the appropriate software installed, you can download article citation data to the citation manager of your choice. Simply select your manager software from the list below and click Download.