Performance-Based Design Optimization of Structures: State-of-the-Art Review
Publication: Journal of Structural Engineering
Volume 150, Issue 8
Abstract
Performance-based design optimization (PBDO) aims to design safe, resilient, and cost-effective structures. Methods used for PBDO have evolved by integrating numerical modeling, performance-based design principles, and optimization algorithms. The PBDO framework enables the design of structures with optimal performance and cost. This paper provides researchers with a comprehensive review of the rapidly growing field of PBDO. The evolution of PBDO methods is discussed with the goal of identifying the challenges that must be addressed in future studies. Knowledge gaps are brought to the forefront to emphasize the need for further investigation that expands on the application of PBDO in structural design. Various deterministic and probabilistic formulations for PBDO of structural systems under seismic and wind loading are reviewed. The formulations encompass one or more objective functions, including the upfront, life-cycle, and repair costs. Furthermore, the paper reviews retrofit design studies that have used PBDO methods. The high computational demand in performing PBDO is identified as the major challenge. Possible approaches to alleviate this and other challenges are discussed.
Introduction
The need to consider postevent functionality in structural design became evident after the 1994 Northridge earthquake, which resulted in approximately $45 billion in direct economic losses (Eguchi et al. 1998). In prescriptive seismic design, the intended level of reliability is not assessed or understood (Spence and Arunachalam 2022). During the early to mid-1990s, the Federal Emergency Management Agency (FEMA) provided funding to the Applied Technology Council (ATC) and the Building Seismic Safety Council (BSSC) to create guidelines for incorporating performance-based design (PBD) into structural engineering practice (Spence and Arunachalam 2022). PBD procedures have been developed to create resilient communities (Ghobarah 2001). In PBD, the aim is to design structures with predictable performance and specific objectives. Examples of PBD-oriented guidelines include ATC 40 (ATC 1996), FEMA 350 (FEMA 2000b), FEMA 356 (FEMA 2000a), and ASCE 41 (ASCE 2017).
Central to the PBD methodology are allowable states of damage conditioned on different hazard levels [ASCE-41 (ASCE 2017)]. Performance objectives are defined as a set of seismic hazard levels, each corresponding to acceptable structural and nonstructural damage-based performance levels [FEMA-356 (FEMA 2000)]. The first generation of performance-based earthquake engineering (PBEE) [e.g., ATC (1996), FEMA 356 (FEMA 2000a), and ASCE 41 (ASCE 2017)] uses discrete performance levels such as operational (OP), immediate occupancy (IO), life safety (LS), and collapse prevention (CP). The performance levels are defined based on engineering demand parameters, such as plastic rotations, axial deformations, and story drifts. However, a limitation of the first-generation PBEE is that the discrete performance levels did not adequately address stakeholders’ concerns, which are usually tied to repair costs, the time required for repair, risks to human life, and environmental impacts.
In 2012, FEMA released guidelines for the next-generation PBEE methodology. FEMA P-58 (FEMA 2012) represents the current state-of-the-art paradigm for PBEE. The next-generation PBEE methodology has been developed to provide a more comprehensive performance measure. FEMA P-58 considers various sources of uncertainty and adopts performance measures that are more meaningful to stakeholders. A probabilistic framework is implemented to predict the potential consequences of earthquakes, including repair costs, repair time, risk to human life, and environmental impacts. In the last few years, there has been a growing interest in implementing the methodology presented in FEMA P-58 for the seismic performance assessment of buildings (e.g., Del Gobbo et al. 2018; Hutt et al. 2019; Shirkhani et al. 2021). Moreover, risk-targeted design has emerged to facilitate the achievement of consistent performance objectives for a diverse building population by establishing hazard maps that are based on uniform risk (Gkimprixis et al. 2019; Iervolino 2023). The impact of seismic design criteria and varying hazard levels across Europe on both the cost and safety considerations has also been studied (Gkimprixis et al. 2020). Risk-targeted seismic design methodologies have also been explored for specific lateral force-resisting systems such as reinforced concrete frames (Franchin et al. 2018; Sinković et al. 2016). In addition, risk-targeted seismic design has been implemented with the goal of minimizing expected monetary losses while ensuring acceptable building collapse safety (Shahnazaryan et al. 2022).
Inspired by the development of performance-based seismic design [ATC-58-2 (ATC 2003); FEMA-356 (FEMA 2000a)], researchers and professionals have adopted PBD methods for other hazards, such as wind (e.g., Ciampoli et al. 2011; Spence and Arunachalam 2022), fire (e.g., Wang et al. 2012), and tsunamis (e.g., Attary et al. 2017). Structures can be designed using performance-based principles to reliably withstand the effects of different natural hazards. However, finding an optimal design using a traditional design procedure is cumbersome. Previous research has documented the benefits of implementing an optimization framework in PBD. For instance, applying optimization methods for the design of steel structures with nonlinear viscous dampers reduced the expected life-cycle costs by 60% compared to nonoptimal designs (Ahadzadeh Kolour et al. 2021). Another study (Hassanzadeh et al. 2024) reported that optimal placement of self-centering connections could reduce the total cost, including the initial construction and expected repair costs, by up to 71% and 61% for 3- and 9-story steel frames with self-centering connections, respectively, compared to nonoptimal frames. Furthermore, design optimization has been shown to reduce maximum acceleration response by up to 22.6% (Xu et al. 2017). When PBD is applied to structures, several design variables, such as the cross-sectional dimensions and configuration of structural elements, must be considered. Given the diversity in the types of design variables, utilizing an optimization algorithm for addressing the complex task of achieving safe and cost-efficient structures is imperative. Optimization techniques have been integrated into the PBD framework in structural engineering over the last two decades. Performance-based design optimization (PBDO) aims to achieve safe, resilient, and cost-effective structures by minimizing one or more objective functions while considering predefined constraints.
Implementing optimization methods in building (e.g., Ganzerli et al. 2000; Gholizadeh et al. 2022b) and bridge (e.g., Franchini et al. 2022; Wen et al. 2021) structural design offers several advantages. Economical and efficient structures are achieved when design optimization is implemented based on objectives such as minimized material usage (e.g., Kaveh et al. 2010), construction costs (e.g., Liu et al. 2005b), or environmental impacts (e.g., Park et al. 2018). Design optimization of high-performance structures can be used to maximize the enhanced behavioral characteristics, such as stiffness (e.g., Lu et al. 2018), strength, ductility, and collapse safety (e.g., Gholizadeh et al. 2022a), while minimizing undesirable effects, such as large drifts (e.g., Cha et al. 2014; De Domenico and Hajirasouliha 2021) and excessive vibrations. Implementing optimization methods in PBD promotes the development of innovative solutions that are not possible using traditional design methods. While designing for higher resilience to natural hazards (e.g., Omidian and Khaji 2022), optimization facilitates the search for more sustainable structural design solutions by minimizing resource consumption and carbon emissions (e.g., Park et al. 2018). Optimization methods also enable automation of the design iteration to find the most promising solutions (e.g., Hassanzadeh et al. 2024). Moreover, PBDO allows for a holistic structural design approach by considering various interdisciplinary criteria, such as those pertaining to structural (e.g., Gholizadeh and Fattahi 2018), civil, architectural (e.g., de Salles et al. 2023), mechanical, and material engineering (e.g., Hassanzadeh and Moradi 2022).
The majority of studies on PBDO have predominantly concentrated on structural building systems (Ganzerli et al. 2000; Zhang and Tian 2019), highlighting a noticeable scarcity of research on the application of PBDO to bridges (Turchetti et al. 2023; Franchini et al. 2022; Wen et al. 2021). In addition to enabling cost-effective designs (Lagaros et al. 2010; Zou and Chan 2005) with enhanced structural performance (Lavan and Dargush 2009), PBDO methods have wide applications in minimizing structural damage (Möller et al. 2009), life-cycle cost (Liu et al. 2004; Wen and Kang 2001a, b), and emissions (Park et al. 2018), while considering deterministic (Ganzerli et al. 2000; Lagaros et al. 2010; Zhang and Tian 2019) and/or probabilistic (Gholizadeh and Aligholizadeh 2019; Gholizadeh and Mohammadi 2017; Khatibinia et al. 2013; Lagaros et al. 2008; Möller et al. 2009, 2015; Yazdani et al. 2017) constraints. In PBDO, deterministic constraints related to geometry, strength, story drift, plastic rotation of structural elements, and strong column–weak beam (SCWB) design (Ganzerli et al. 2000; Zou et al. 2007a; Zou and Chan 2005) can be defined. Within this framework, it is possible to incorporate probabilistic constraints related to structural reliability and failure probability (Spence and Gioffrè 2012; Zacharenaki et al. 2013; Zhang and Foschi 2004). Moreover, various optimization strategies, such as gradient-based (Mirfarhadi et al. 2021) and metaheuristic algorithms (Liu et al. 2003), analysis/redesign procedures (Bai et al. 2020; De Domenico et al. 2019; Levy and Lavan 2006; Shmerling et al. 2018), and uniform damage distribution methods (Asadi and Hajirasouliha 2020), can be used. It is noteworthy that PBDO predates the emergence of metaheuristic methods (Ganzerli et al. 2000). However, the latter has significantly contributed to the popularity and widespread application of PBDO.
In PBDO, different categories of optimization, including size (Chan and Zou 2004; Gholizadeh 2015; Zou and Chan 2005) and topology (Hassanzadeh and Gholizadeh 2019; Liu et al. 2005b), can be implemented. Size optimization determines the most suitable dimensions of structural elements, such as beams, columns, and braces, to achieve minimum cost under the relevant loading. Topology optimization identifies the most efficient layout or distribution of materials in a given design space, subject to specific constraints and loading. Fig. 1 presents examples of topology optimization applications in building structures. By combining size and topology optimization methods, safe and cost-effective structures can be designed (Hassanzadeh and Gholizadeh 2019). While the majority of research has focused on the optimization of regular structures, a limited number of studies have addressed design optimization of irregular structures (e.g., Alkhatib et al. 2022; Marzok and Lavan 2023a, b). Recent studies (Hassanzadeh and Moradi 2022) have applied performance-based optimization to earthquake-resilient steel frames using shape memory alloy (SMA) materials. The optimal placement of SMA braces or connections minimizes the use of SMAs, which are expensive but needed to provide self-centering capability (i.e., returning the structure to its original position). In addition, optimization methodologies have been used in the design and assessment of self-centering steel moment frames (e.g., Idels and Lavan 2023) and rocking steel braced frames (Burton et al. 2019; Marzok and Lavan 2022, 2023a, b).
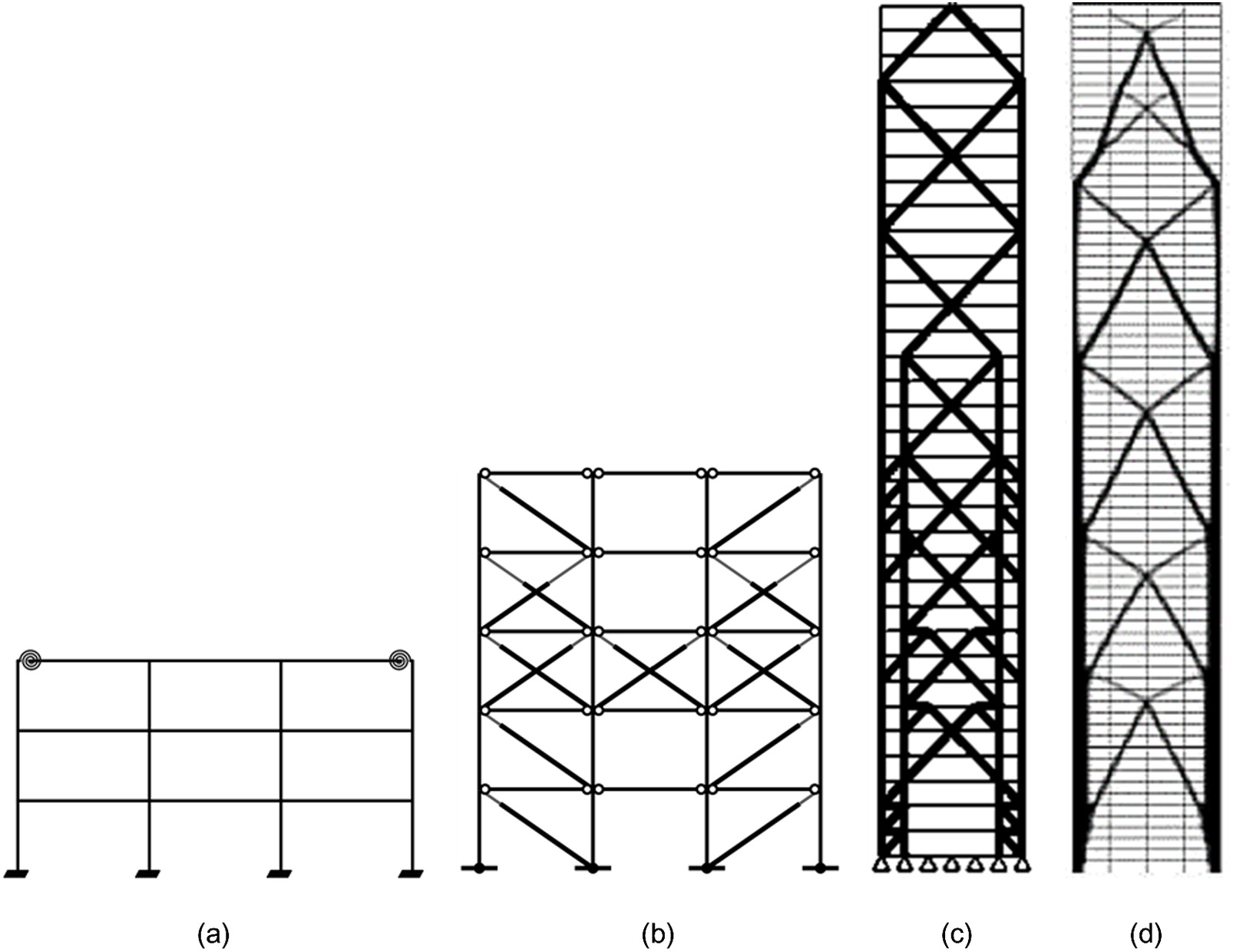
In the last two decades, a few literature review studies have surveyed the application of PBDO in structural engineering. However, a comprehensive review that covers the most recent research is lacking. A review paper by Liang (2007) presented a comprehensive overview of advancements in automated PBD techniques, with a specific focus on topology optimization of continuum structures. The latest PBDO review study was conducted by Fragiadakis and Lagaros (2011). They presented an overview of deterministic and probabilistic frameworks for seismic design optimization of a steel braced frame using a metaheuristic algorithm. Other review papers (Liang 2007; Fragiadakis and Lagaros 2011) primarily focused on size or topology optimization of seismic systems. In contrast, this review study has a broader scope that includes various types of optimization methods applied to structural systems under seismic and wind loading.
This paper presents a comprehensive review of PBDO in structural engineering, covering journal papers since the year 2000. The goal of this review is to answer the following questions: (1) What are the latest developments? (2) What are the challenges in adopting PBDO in practice? (3) What are the solutions to address the challenges? and (4) How can PBDO methodologies help professional engineers create resilient and sustainable structures? The paper is divided into three main sections: “PBDO with Cost Consideration,” “PBDO for Retrofit Design of Structures,” and “Approaches to Reducing Computational Time.” In the “PBDO with Cost Consideration” section, the initial, life-cycle, and Park–Ang repair costs are discussed. In all three sections, the background and potential research gaps are examined. The main findings from this review study are also discussed. Fig. 2 provides a summary of prior PBDO studies.
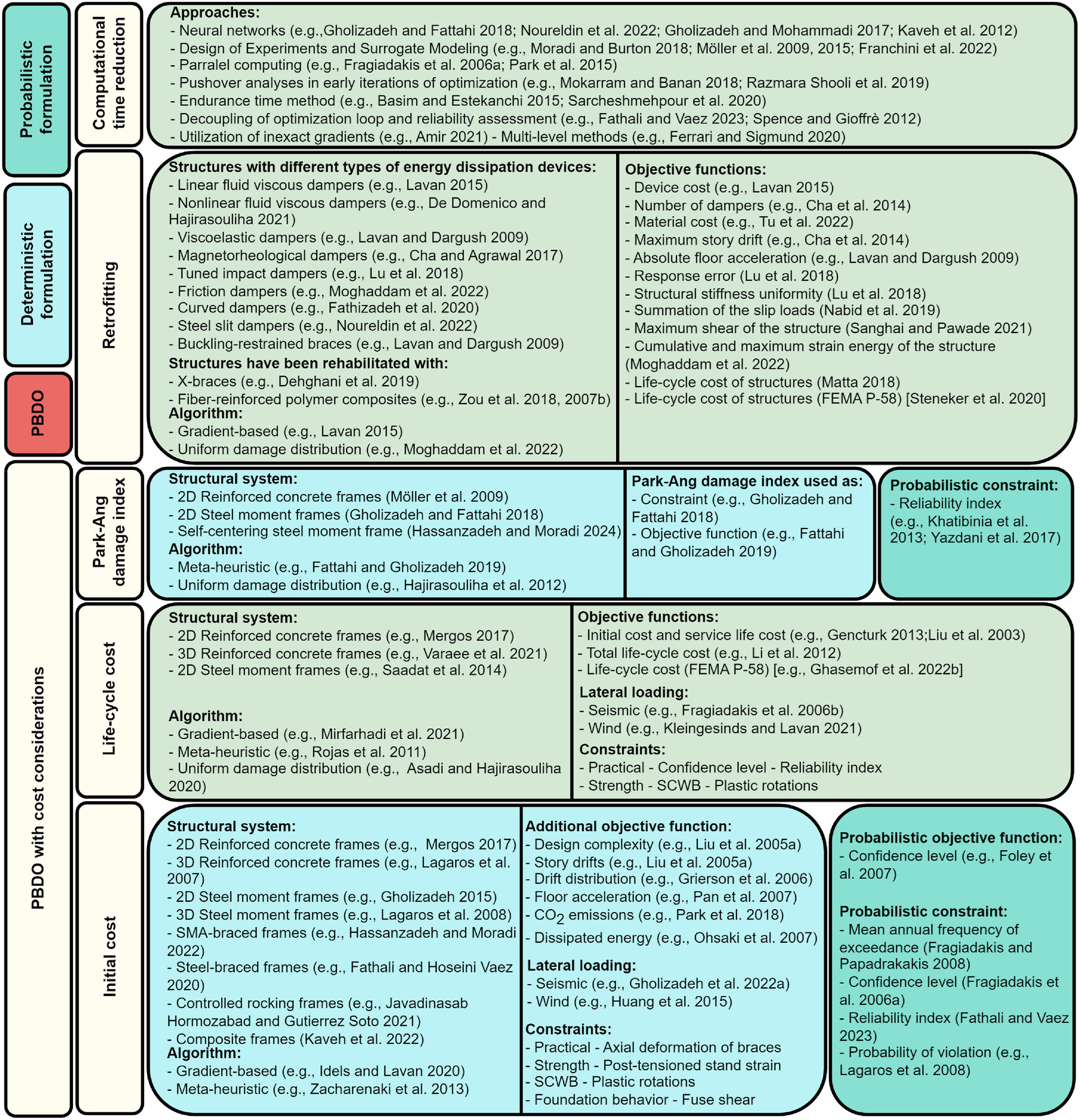
Literature Review Methodology
This study conducts a systematic review of the PBDO literature. Related studies published as recently as January 2024 were found by searching for relevant keywords in Scopus and major publishers, including ASCE, Elsevier, SAGE, Springer, Taylor and Francis, and Wiley. In addition, backward and forward citation procedures were used for the comprehensive literature review. The search keywords included “performance-based design,” “optimization,” “optimal,” “structure,” and/or “life-cycle cost.”
Specific search criteria were used to determine which papers should be included or excluded from the study. Duplicates and non-English records were removed from the collected files. Journal papers were excluded when (1) the research focus was not related to PBDO, (2) the paper was not peer-reviewed, (3) the complete paper was not accessible, and (4) the main findings in the paper were already discussed in prior studies. Following the delimiting criteria, 165 out of 341 papers were selected. The oldest and latest studies were published in 2000 and 2024, respectively. Previous research was classified based on four types of problem formulations, including PBDO considering initial cost, life-cycle cost, repair cost, and optimal retrofit design of structures. Several papers were reviewed strategically to provide researchers with a focused and in-depth understanding of the diverse subtopics within the broader PBDO domain. This organization enables the review of studies with cost considerations, retrofit design optimization strategies, and techniques for enhancing computational efficiency in PBDO. The publication trend between 2000 and 2024 is visually shown in Fig. 3. Overall, the number of published papers increased by 367% from 2000 to 2022. Since 2015, there has been a noticeable rise in utilizing PBDO methodologies for retrofit design. In addition, there has been a growing trend in recent years where optimization studies increasingly incorporate life-cycle cost in the objective function.

PBDO with Cost Considerations
PBDO studies may include the initial (Chan and Zou 2004; Liu et al. 2005a), life-cycle (Ghasemof et al. 2021; Taflanidis and Beck 2009; Wen and Kang 2001a), and repair cost (Fattahi and Gholizadeh 2019; Hajirasouliha et al. 2012; Kaveh et al. 2015) as objective functions, along with predefined structural constraints. The following subsections review PBDO studies that consider different cost types. Fig. 4 shows the flowcharts for PBDO with two types of costs: initial and life-cycle. For life-cycle cost, the FEMA P-58 methodology is depicted in Fig. 4. PBDO with repair cost that incorporates the Park–Ang damage index as a constraint distinguishes it from the initial cost optimization problems. For PBDO problems incorporating repair costs using the Park–Ang damage index as an objective function, an additional constraint related to the Park–Ang damage index is included along with other checks. In the FEMA P-58 methodology, evaluating the seismic performance of buildings involves a comprehensive assessment and quantification of performance metrics such as casualties, repair cost, and repair time. In optimization studies, structural design checks are defined as constraints within the optimization process. The design variables are adjusted during each iteration to minimize the objective function while adhering to the specified constraints. Achieving a feasible design implies that all constraints are satisfied. Examples of such constraints include geometry, strength, story drift, plastic rotation of structural elements, SCWB, and PBD checks. The constraint for story drift is presented here as an examplewhere and are the peak story drift and the allowable story drift at the performance level, pl, respectively. The peak story drift is calculated using an appropriate structural analysis routine during the optimization process. The allowable story drift is defined according to design codes and standards.
(1)
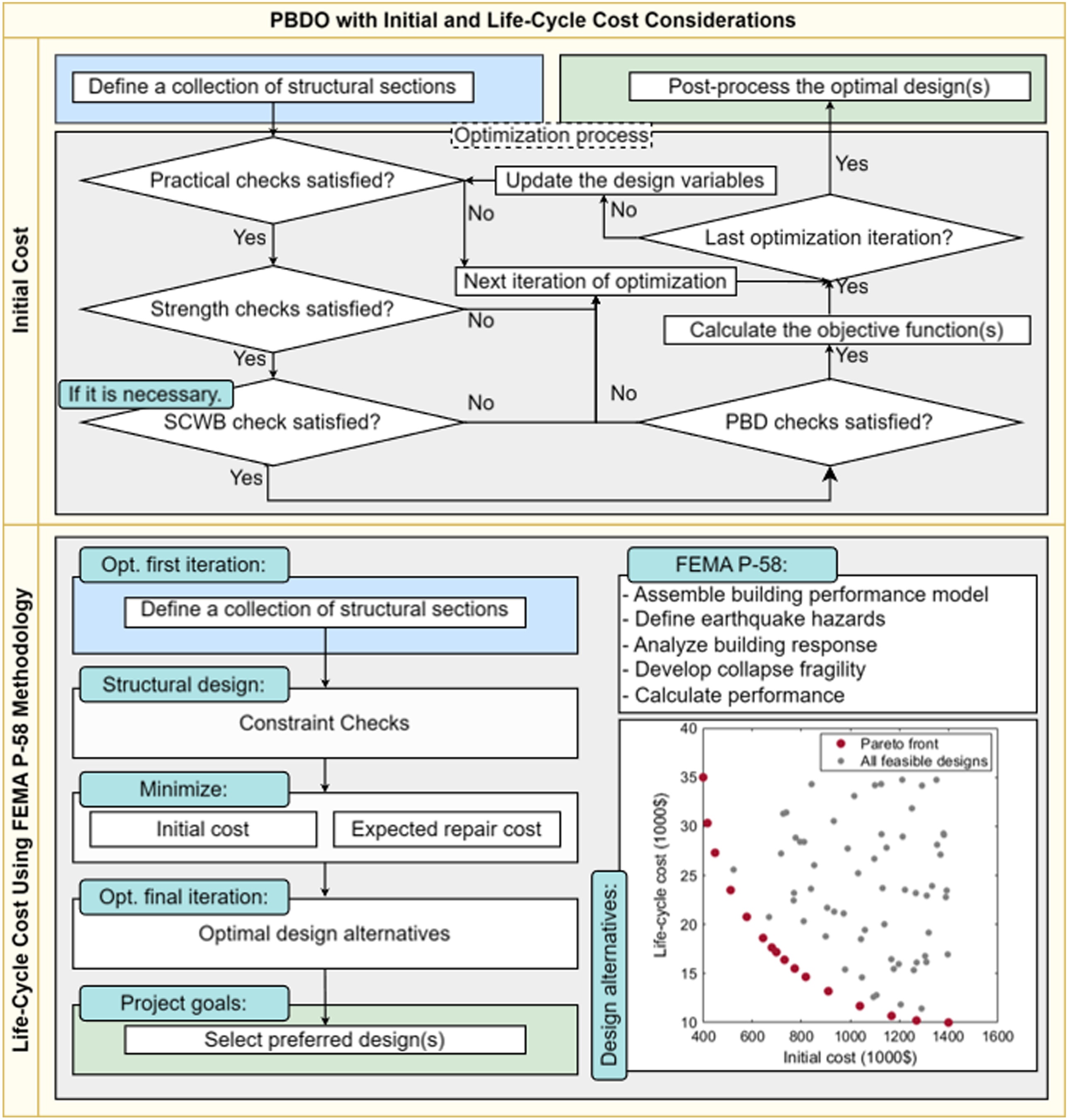
Initial Cost
Table 1 summarizes studies that conducted PBDO of seismic structural systems with initial cost considerations.
Structural system | References | Number of stories | Design variables | Objective function(s) | Constraint(s) | Algorithm |
---|---|---|---|---|---|---|
Reinforced concrete frame | Ganzerli et al. (2000) | 1 | Size of elements | Initial cost | Practical checks | Design optimization tool |
Plastic rotations | ||||||
Chan and Zou (2004) | 10 | Size of elements | Initial cost | Practical checks | Optimality criteria | |
Story drifts | ||||||
Fragiadakis and Papadrakakis (2008) | 2 | Size of elements | Initial cost | Practical checks | Metaheuristic | |
Strength checks | ||||||
Story drifts | ||||||
Mean annual frequency of exceedance | ||||||
Mergos (2017) | 1 and 4 | Size of elements | Initial cost | Practical checks | Metaheuristic | |
Strength checks | ||||||
Story drifts and plastic rotations | ||||||
Park et al. (2018) | 4 and 10 | Size of elements | Initial cost | Practical checks | Metaheuristic | |
emissions | Strength checks | |||||
Coefficient of variation of story drifts | ||||||
Story drifts | ||||||
3D reinforced concrete frame | Lagaros and Papadrakakis (2007) | 2 | Size of elements | Initial cost | Story drifts | Metaheuristic |
Story drifts | ||||||
Steel moment frame | Liu et al. (2005a) | 5 | Size of elements | Initial cost | — | Metaheuristic |
Design complexity | ||||||
Story drifts | ||||||
Fragiadakis et al. (2006a) | 9 | Size of elements | Initial cost | Confidence level | Metaheuristic | |
Grierson et al. (2006) | 9 | Size of elements | Initial cost | — | Optimality criteria | |
Drift distribution | ||||||
Lagaros et al. (2006) | 6 | Size of elements | Initial cost | Strength and SCWB checks | Metaheuristic | |
Story drifts and plastic rotations | ||||||
Foley et al. (2007) | 3 | Size of elements | Initial cost | SCWB | Metaheuristic | |
Confidence level | Confidence level | |||||
Gholizadeh and Mohammadi (2017) | 3 and 10 | Size of elements | Initial cost | Practical checks | Metaheuristic | |
Strength checks | ||||||
Story drifts | ||||||
Reliability index | ||||||
Ohsaki et al. (2007) | 5 | Size of elements | Initial cost | Story drifts | Metaheuristic | |
Dissipated energy | ||||||
Pan et al. (2007) | 4 and 8 | Size of elements | Initial cost | Story drifts | Nonlinear programming method | |
Floor acceleration | ||||||
Rojas et al. (2007) | 2 | Size of elements | Initial cost | SCWB | Metaheuristic | |
Confidence level | ||||||
Liu et al. (2013) | 4 | Size of elements | Initial cost | Strength checks | Metaheuristic | |
Drift distribution | Confidence level | |||||
Story drifts | ||||||
Idels and Lavan (2020) | 2, 5, and 9 | Size of elements | Initial cost | Story drifts | Gradient-based | |
Zacharenaki et al. (2013) | 3 and 9 | Size of elements | Initial cost | Practical checks | Metaheuristic | |
Strength checks | ||||||
Mean annual frequency of exceedance | ||||||
Gholizadeh (2015) | 3, 6, and 12 | Size of elements | Initial cost | Strength checks | Metaheuristic | |
Story drifts | ||||||
Fathizadeh et al. (2021) | 6 and 9 | Size of elements | Initial cost | Practical checks | Metaheuristic | |
Strength and SCWB checks | ||||||
Story drifts and Plastic rotations | ||||||
Foundation behavior | ||||||
Gholizadeh et al. (2022a) | 3, 6, and 12 | Size of elements | Initial cost | Practical checks | Metaheuristic | |
Strength and SCWB checks | ||||||
Confidence level | ||||||
Story drifts and Plastic rotations | ||||||
Collapse safety | ||||||
Fathali and Vaez (2023) | 3 and 9 | Size of elements | Initial cost | Practical checks | Metaheuristic | |
Strength checks | ||||||
Story drifts and Plastic rotations | ||||||
Reliability index | ||||||
3D steel moment frame | Lagaros et al. (2008) | 7 | Size of elements | Initial cost | Strength and SCWB checks | Metaheuristic |
Story drifts | ||||||
Probability of violation | ||||||
Braced frame | Gholizadeh and Poorhoseini (2016) | 6, 9, and 12 | Size of elements | Initial cost | Practical checks | Metaheuristic |
Brace Topology | Strength checks | |||||
Story drifts and Plastic rotations | ||||||
Axial deformation of braces | ||||||
Fathali and Hoseini Vaez (2020) | 3 and 6 | Size of elements | Initial cost | Practical checks | Metaheuristic | |
Drift distribution | Strength checks | |||||
Story drifts | ||||||
Practical checks | ||||||
Strength checks | ||||||
Story drifts and plastic rotations | ||||||
Axial deformation of braces | ||||||
Hassanzadeh and Gholizadeh (2019) | 5, 10, and 15 | Size of elements | Initial cost | Practical checks | Metaheuristic | |
Brace Topology | Strength checks | |||||
Story drifts and plastic rotations | ||||||
Axial deformation of braces | ||||||
Hassanzadeh and Moradi (2022) | 5 and 10 | Size of elements | Initial cost | Strength checks | Metaheuristic | |
Brace Topology | Story and residual story drifts | |||||
Fuse shear deformation | ||||||
Post-tensioned stand strain | ||||||
Controlled rocking frame | Javadinasab Hormozabad and Gutierrez Soto (2021) | 6 | Size of elements | Initial cost | Strength checks and Story drifts | Gradient-based |
Composite frame | Kaveh et al. (2022) | 8 and 20 | Size of elements | Initial cost | Metaheuristic |
Deterministic Formulation
The initial cost optimization in a PBD context focuses on minimizing construction costs without considering potential repair costs over the service life of the building. The initial cost optimization in a deterministic PBD framework can be expressed as follows:where is the initial cost of structural members; are the deterministic constraints; and is the number of constraints.
(2)
Over the last two decades, numerous studies have developed and implemented PBDO methodologies to identify optimal earthquake-resistant systems with minimal structural cost (e.g., Ganzerli et al. 2000; Hassanzadeh and Gholizadeh 2019). Ganzerli et al. (2000) developed a size optimization methodology for reinforced concrete frames under seismic loading in the context of PBD using a design optimization tool (Vanderplaats 1995). Their research laid the foundation for subsequent studies in PBDO. PBD methodologies were also used to minimize the cost of several different types of structural systems, including reinforced concrete frames (Ganzerli et al. 2000; Lagaros et al. 2010; Zhang and Tian 2019), steel moment frames (Foley et al. 2007; Idels and Lavan 2020; Vaez et al. 2024), steel braced frames (Fathali and Hoseini Vaez 2020; Wang et al. 2020), concrete shear walls (Huang et al. 2015), and self-centering frames (Hassanzadeh and Moradi 2022; Javadinasab Hormozabad and Gutierrez Soto 2021). Recently, PBDO methodologies have been implemented to design unsymmetrical structures in the PBD context (Hoseini Vaez et al. 2022).
The design variables in the initial cost optimization of reinforced concrete frames within a PBD framework can include the cross-sectional area of the concrete sections (Chan and Zou 2004), the area of steel reinforcement, or a combination of both (Ganzerli et al. 2000; Lagaros et al. 2010; Mergos 2017, 2018; Zou and Chan 2005). In Zhang and Tian (2019), the relative flexural stiffness and strength of reinforced concrete sections were taken as design variables. In the optimization of steel frames, the design variables can be categorized into two primary groups: size and topology design variables. Specifically, the cross-sectional dimensions of beams, columns (Fragiadakis et al. 2006a), and braces (Gholizadeh et al. 2022b) fall within the size variables, while the positioning of braces is regarded as a topology variable (Gholizadeh and Ebadijalal 2018; Gholizadeh and Poorhoseini 2016; Wang et al. 2020). Reinforced concrete structures, due to their complex composition and detailing, require a considerably larger number of design variables compared to steel frame structures, which complicates the problem formulation (Mergos 2017).
In recent years, SMA materials have emerged as a promising solution for reducing or eliminating residual deformation in structures following severe earthquakes (Hassanzadeh and Moradi 2022; Leon et al. 2001; Ocel et al. 2004). Despite their potential benefits, one of their drawbacks is the relatively high cost of the material. In Hassanzadeh and Moradi (2022), a topology PBD optimization methodology was applied to reduce the amount of SMA in low-damage steel braced frames. A range of size and topology design variables, including the cross sections of columns and SMA braces, as well as the location of SMA braces, were considered. Optimization methodologies have also been used for the PBD of controlled rocking braced frames (Javadinasab Hormozabad and Gutierrez Soto 2021).
The initial cost optimization in the PBD context considers several constraints, including, among others, practical, strength-related, SCWB (Fragiadakis and Papadrakakis 2008), and confidence levels (Alimoradi et al. 2007; Rojas et al. 2007). Practical constraints are based on the dimensions of the structural members, which are evaluated from a constructability standpoint (Chan and Zou 2004; Ganzerli et al. 2000; Hassanzadeh and Moradi 2022). Strength-related constraints are used to check the demand–capacity ratio for structural elements following conventional seismic design codes [ANSI/AISC 360-16 2016 (AISC 2016); Gholizadeh and Moghadas 2014; Lagaros et al. 2006, 2010)]. PBD constraints in structural optimization can be defined based on engineering demand parameters, such as plastic rotation (Ganzerli et al. 2000; Lagaros et al. 2006), axial deformation of structural members (Hassanzadeh and Gholizadeh 2019; Hassanzadeh and Moradi 2022), and story drifts (Gholizadeh and Poorhoseini 2016; Kaveh et al. 2010; Lagaros et al. 2006; Zou and Chan 2005) at different performance levels.
Optimization procedures such as metaheuristic (Fathali and Hoseini Vaez 2020; Kaveh and Nasrollahi 2014; Liu et al. 2005a; Yaghoobi et al. 2023) and gradient-based algorithms (Fu et al. 2018; Huang et al. 2015; Idels and Lavan 2020; Mergos 2017; Suksuwan and Spence 2018), and the uniform distribution of the deformation technique (Dong et al. 2023), have been implemented to design cost-effective structures. Evolutionary algorithms and gradient-based approaches are two different optimization methods that can be used in PBDO. Evolutionary strategies, such as genetic algorithms (GAs) and evolutionary programming (EP), maintain and iteratively evolve a population of solutions (Lagaros et al. 2002). In contrast, gradient-based approaches, such as gradient descent and quasi-Newton methods, rely on gradient information to iteratively update the solution. Moreover, evolutionary algorithms search for promising regions in a population-based manner, while gradient-based approaches explore the search space in a deterministic, local manner guided by gradient information. As a result, evolutionary algorithms are more suitable for highly complex optimization problems (van de Lindt and Dao 2007). On the other hand, gradient-based approaches are suitable for optimization problems with continuously differentiable objective functions. Compared to gradient-based solutions, metaheuristic algorithms are simpler because they do not require the gradients of constraints (Fragiadakis et al. 2006a). Evolutionary algorithms are powerful in global optimization tasks, providing reliable solutions that are robust to uncertainties, while gradient-based approaches are popular for their computational efficiency (Suksuwan and Spence 2019) and rapid convergence (Salajegheh et al. 2022). In a simple linear optimization problem, the computational efficiency of a gradient-based algorithm was found to surpass that of heuristic algorithms by 56% (Krogh et al. 2017). This disparity is expected to increase significantly in more complex optimization problems, particularly when the search involves performing response history analyses. Moreover, sequential linear programming (SLP) was found to reduce the number of response history analyses (Pollini et al. 2017). Achieving a satisfactory solution using a GA necessitates using a large population, 350 individuals, and conducting five optimization iterations. This translates to performing 350 response history analyses for each of the five optimization iterations. Pollini et al. (2018) converted their initial mixed-integer problem formulation into a continuous one. This conversion enables the nonlinear programming problem to be solved using a gradient-based SLP, which significantly reduces the computational burden.
Metaheuristic algorithms have been used in the topology optimization of steel braced frames in the PBD context with size, strength-related, and PBD constraints (Ebadijalal and Shahrouzi 2022; Eftekhar et al. 2022; Gholizadeh et al. 2022b; Gholizadeh and Ebadijalal 2018; Gholizadeh and Poorhoseini 2016; Karimi and Hoseini Vaez 2019; Kaveh et al. 2022).
Analysis procedures, such as the virtual work principle, pushover analysis, and nonlinear response history analysis (NRHA), have been used to evaluate the performance of optimized structures. While pushover analyses are faster (Chan and Zou 2004; Kaveh et al. 2010), NRHAs are preferred due to their ability to capture the dynamic response of the structure (Foley et al. 2007; Lagaros et al. 2006, 2010). The principle of virtual work has been utilized to assess the elastic response in the optimization process (Chan and Zou 2004). The response prediction accuracy is contingent on the modeling characteristics, such as the inclusion of soil–structure interaction (Fathizadeh et al. 2021; Gholizadeh et al. 2023) and the element and material nonlinearity. Incremental dynamic analyses are needed when assessing the collapse safety of optimal building frames per FEMA P695 (Fattahi and Gholizadeh 2019; Hassanzadeh and Moradi 2022). In Gholizadeh et al. (2022a), the collapse margin ratio was considered as a constraint in the PBDO. In gradient-based optimization methodologies, discrete design variables may need to be replaced by continuous approximations, which enables an analytical assessment of the gradients (Idels and Lavan 2021a; Marzok and Lavan 2022).
Several conflicting criteria can be addressed in PBDO [e.g., for design under earthquake (Grierson et al. 2006; Lagaros and Papadrakakis 2007) and wind (Kleingesinds and Lavan 2022)]. Multiobjective optimization methodologies have been implemented to make a trade-off between different conflicting objective functions. In addition to the initial cost, objective functions have included peak story drift (Ayyash and Hejazi 2023; Grierson et al. 2006; Lagaros et al. 2007), story drift variation (Liu et al. 2013), absolute floor acceleration (Pan et al. 2007), post–elastic ductility demand along the structure’s height (Xu et al. 2006), and emission (Park et al. 2018).
The response of structures under wind action is different from earthquakes. In Huang et al. (2015), a PBDO methodology was proposed for designing tall buildings subjected to wind loading. The initial cost was considered as the objective function, and the constraints included the elastic and plastic story drift, peak modal and transient acceleration, dynamic serviceability, and the section size of steel and concrete members. Nonlinear pushover analysis has also been implemented to find the structural response in the optimization process. In Fu et al. (2018), the occupant comfort level was assessed based on the modal acceleration response of buildings.
Probabilistic Formulation
PBD methodologies can consider different sources of uncertainty (Alimoradi et al. 2007; Foley et al. 2007; Rastegaran et al. 2022) that arise from earthquake ground motions, structural geometry, materials, and numerical models (Fragiadakis and Lagaros 2011; Rastegaran et al. 2022). Reliability-based design optimization has been developed to consider unavoidable uncertainties as constraints. A reliability-based design optimization problem can be formulated as follows (Zacharenaki et al. 2013):where is a probabilistic constraint of the th limit-state; and are limit-state probability of exceedance and preset thresholds; is the number of discrete constraints; and is the number of probabilistic constraints, respectively.
(3)
The limit-state probability of exceedance is calculated based on the total probability theorem as follows (Fragiadakis and Lagaros 2011):where is the limit-state mean annual frequency (MAF); EDP is the engineering demand parameter; is the intensity measure; and represents the probability that an engineering demand parameter will exceed the threshold value associated with the limit state (edp), given a particular intensity measure value ().
(4)
In complex numerical models, Monte Carlo Simulation (MCS) can be used to determine probabilistic constraints (Gholizadeh and Aligholizadeh 2019; Gholizadeh and Mohammadi 2017; Lagaros et al. 2008). Moreover, to consider the various sources of uncertainty in the optimization, a framework has been proposed whereby optimally designed frames meet specified performance objectives with a predetermined confidence level following FEMA 350 [Fattahi and Gholizadeh 2019; FEMA-350 2000 (FEMA 2000b); Fragiadakis et al. 2006a]. Previous research studies (Alimoradi et al. 2007; Foley et al. 2007) have presented a framework for minimizing the initial cost while maximizing the structural performance confidence level. Furthermore, predefined confidence level constraints for nonstructural elements have been considered in PBDO following HAZUS (FEMA 2013; Rojas et al. 2007).
Reliability-based design optimization frameworks have also been proposed for structures subjected to wind loads (Spence and Gioffrè 2012; Spence and Kareem 2014). The constraints were the deterministic serviceability drift performance, probabilistic occupant comfort acceleration performance, and geometry checks (Huang et al. 2012). Some studies have represented these constraints in terms of failure probabilities (Deng et al. 2019). In Bobby et al. (2014), steel braced frames subjected to wind loading were optimized considering topology-based design variables. The MCS method was used to define probabilistic PBD constraints. Bobby et al. (2014) have also proposed a methodology to perform multihazard topology optimization considering seismic and wind actions. In another study (Bobby et al. 2016), fragility curves were utilized to define probability constraints.
Discussion
Previous research on initial cost optimization within the PBD framework has predominantly focused on two-dimensional (2D) frames (e.g., Fathali and Hoseini Vaez 2020; Ganzerli et al. 2000). This limits the relevance of optimization research to structural design practice. This limitation can be addressed by developing practical PBDO software tools (Lagaros 2014).
Metaheuristic algorithms can be implemented to improve the practicality of the optimization methodologies (Gholizadeh and Ebadijalal 2018). However, the use of metaheuristic algorithms in optimization requires computational resources. Recommendations to address this challenge are presented in the “Approaches to Reducing Computational Time” section of this paper. Optimization studies also need to include the life-cycle and repair costs of the structure to broaden the optimization benefits and its applications in practice, as detailed in the next section.
In addition to conventional structural systems, PBDO can be implemented for the design of novel systems, such as low-damage self-centering structures equipped with SMAs. PBDO can be used to promote the application of novel structural systems by developing guidelines for their effective design (Hassanzadeh and Moradi 2022).
Safety is paramount in structural design. Considering collapse safety indices in the optimization process is essential in achieving a trade-off between safety and initial cost (Fattahi and Gholizadeh 2019; Hassanzadeh and Moradi 2022). Further, additional research is needed in the area of multihazard PBDO, particularly considering both wind (Huang et al. 2015; Suksuwan and Spence 2018) and seismic loading. Addressing these needs and challenges will lead to more efficient and effective structural design methodologies that can be implemented in practice.
Reliability-based design optimization problems often rely on MCS techniques to estimate the probability of failure. While this method has proven effective, its computational expense is significant, which limits its practicality (Gholizadeh and Aligholizadeh 2019; Gholizadeh and Mohammadi 2017; Lagaros et al. 2008). The uncertainty in the connection model, mass, and damping ratio should also be considered in probabilistic assessments (Liu et al. 2013). Only a few structural systems have been optimized using reliability-based design principles (Fragiadakis and Papadrakakis 2008; Gholizadeh and Aligholizadeh 2019; Gholizadeh and Mohammadi 2017; Zhang and Foschi 2004). Other structures, such as steel braced frames, steel shear wall systems, and low-damage self-centering systems, can also be designed using reliability-based design optimization.
Life-Cycle Cost
Several studies have considered building life-cycle cost as an objective function in design optimization. These studies are summarized in Table 2, where only size-related design variables have been considered.
Structural system | References | Number of stories | Objective function(s) | Constraint(s) | Algorithm |
---|---|---|---|---|---|
Reinforced concrete frame | Esteva et al. (2002) | 10 | Total life-cycle cost | — | Not stated. |
Zou et al. (2007a) | 10 | Initial cost | Practical checks | Optimality criteria | |
Service life cost | Story drifts and Plastic rotations | ||||
Lagaros and Fragiadakis (2011) | 3 and 6 | Total life-cycle cost | Strength checks | Metaheuristic | |
Gencturk (2013) | 2 | Initial cost | — | Metaheuristic | |
Service life cost | |||||
Möller et al. (2015) | 3 | Total life-cycle cost | Reliability index | Metaheuristic | |
Asadi and Hajirasouliha (2020) | 5, 8, and 12 | Total life-cycle cost | — | Uniform damage distribution | |
Razavi and Gholizadeh (2021) | 3, 6, and 12 | Total life-cycle cost | Practical checks | Metaheuristic | |
Strength checks and SCWB | |||||
Story drifts | |||||
3D reinforced concrete frame | Mitropoulou et al. (2011) | 5 and 8 | Initial cost | Strength checks | Metaheuristic |
Service life cost | Story drifts | ||||
Varaee et al. (2021) | 4 | Total life-cycle cost (FEMA P-58) | Practical checks | Metaheuristic | |
Strength checks and SCWB | |||||
Steel moment frame | Wen and Kang (2001a) | 9 | Total life-cycle cost | — | Not stated. |
Liu et al. (2003) | 5 | Initial cost | Strength checks | Metaheuristic | |
Service life cost | |||||
Fragiadakis et al. (2006b) | 10 | Initial cost | Strength checks and SCWB | Metaheuristic | |
Service life cost | |||||
Li et al. (2012) | 9 | Total life-cycle cost | Story drifts | Adaptive simulated annealing | |
Rojas et al. (2011) | 3 | Initial cost | Confidence level | Metaheuristic | |
Service life cost | Story drifts | ||||
Kaveh et al. (2014) | 10 | Initial cost | Practical checks | Metaheuristic | |
Service life cost | Strength checks and SCWB | ||||
Saadat et al. (2014) | 3 | Initial costService life cost | Confidence level | Metaheuristic | |
SCWB | |||||
Basim and Estekanchi (2015) | 5 | Initial cost | Practical checks | Metaheuristic | |
Service life cost | Strength checks and SCWB | ||||
Story drifts | |||||
Mirfarhadi et al. (2021) | 2, 4, 8, and 12 | Total life-cycle cost (FEMA P-58) | Strength checks and SCWB | Gradient-based | |
Story drifts | |||||
Ghasemof et al. (2022b) | 3, 6, and 9 | Initial cost | Practical checks | Metaheuristic | |
Life-cycle cost (FEMA P-58) | Strength checks and SCWB | ||||
Story drifts |
Previous Research and Current Practice
PBDO can be formulated to minimize the total life-cycle cost over the service life of a building. Life-cycle cost depends on factors such as the building service life, death and injury costs, uncertainty in the structural capacity, and the assumed discount rate (Wen and Kang 2001a, b). To find the most influential parameters in the life-cycle cost of buildings, a full factorial design has been used (Mousazadeh et al. 2020). Fig. 5 presents a simplified illustration of the expected life-cycle cost, which shows the increase in the initial cost of the structure and the reduction in expected repair cost, ultimately leading to designs that minimize the life-cycle cost.
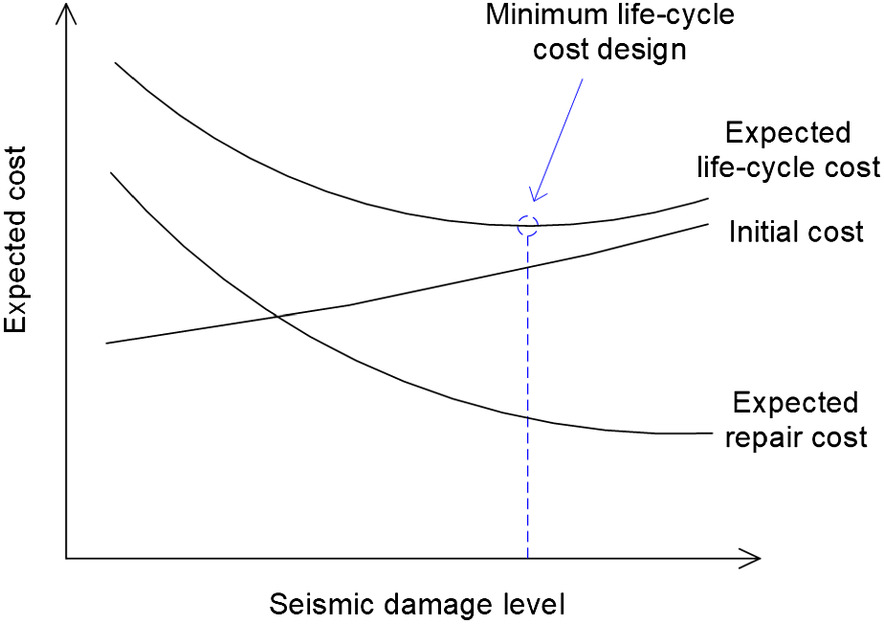
A life-cycle cost optimization methodology was proposed in Wen and Kang (2001a, b) and applied to a 9-story steel office building subjected to earthquake and wind loading. Other researchers (Fragiadakis et al. 2006b; Kaveh et al. 2012; Liu et al. 2003) have utilized the same expected life-cycle cost formulation. The life-cycle cost calculation considering damage states can be computed as follows (Wen and Kang 2001a, b):where is the th limit-state cost; is the probability of the th damage state being violated; and are the general and annual probability of exceeding the relevant engineering demand parameter; is the annual discount rate; is the annual occurrence rate of significant earthquakes modeled as a Poisson process; is the service life of the structure; and and are determined by fitting a curve to known values of for hazard levels and the associated with the considered performance level. Table 3 provides the drift-based, , damage states that have been used for different types of structural systems (Jiang et al. 2020; Lagaros and Fragiadakis 2011; Liu et al. 2004; Wen and Kang 2001b).
(5)
(6)
(7)
(8)
Table 4 summarizes the floor acceleration–based limit-states that have been considered in life-cycle cost calculations (Basim and Estekanchi 2015; Lagaros and Magoula 2013; Shin and Singh 2017). The maximum floor acceleration, , can be used to assess the losses associated with building contents (e.g., furniture and equipment).
To calculate limit-state probabilities, designer-specified confidence levels (CLs) have been utilized (Liu 2005; Lagaros and Fragiadakis 2007) (as shown in Table 5). This methodology can be implemented to consider the uncertainty and randomness per FEMA 350 (FEMA 2000b).
In life-cycle cost optimization problems, both single objective or multiobjective functions can be considered. Several studies have used the summation of initial and service life costs as the total life-cycle cost in the optimization process (Esteva et al. 2002; Matta 2018; Wen and Kang 2001a). In life-cycle cost optimization, considering total cost as the objective function leads to a single optimal design. However, investment decision makers aim to choose the best design from a range of options by considering their personal risk tolerance and the project’s level of importance. This can be achieved by implementing multiobjective optimization techniques (Ghasemof et al. 2021). Some studies (Fragiadakis et al. 2006b; Liu et al. 2003; Mitropoulou et al. 2011) have considered initial and service-life costs as separate objective functions because they are often conflicting objectives.
The seismic performance of the entire building, along with its contents, is notably influenced by the structural system. In an office building, the structural system constitutes 20% of the overall building expenditure (Mayes et al. 2013). Therefore, it is imperative that structural engineers adopt a broader perspective when evaluating seismic performance, considering both structural and nonstructural elements of a building rather than focusing only on structural elements and life-safety considerations. Prior studies have considered nonstructural damage in the life-cycle cost calculation (Rojas et al. 2011; Saadat et al. 2014). Damage to nonstructural elements can be defined using fragility functions (Rojas et al. 2011), which are used in life-cycle cost calculations (Jiang et al. 2020; Shin and Singh 2014b). Some studies calculated the life-cycle cost based on HAZUS (El-Khoury et al. 2018; Ghasemof et al. 2021). This methodology considers five damage states: intact, light, moderate, extensive, and complete.
The consideration of cumulative low-cycle fatigue damage is important in the seismic vulnerability assessment of steel moment frames subjected to major earthquakes. Ghaderi and Gholizadeh (2021) evaluated the mainshock–aftershock low-cycle fatigue damage in optimal steel moment frames. Frames optimized for life-cycle cost were found to be safer against low-cycle fatigue damage compared to those optimized solely for the initial cost.
A few studies (Turchetti et al. 2023) have applied PBDO to the design of bridges. Component-level fragility functions were generated in Wen et al. (2021) to determine the total repair cost in the optimization of damping devices for cable-stayed bridges. In Franchini et al. (2022), parameterized fragility functions were generated and used for sensitivity analysis and optimization of cable-stayed bridges. A probabilistic PBDO was used in Li and Conte (2018) to design seismic isolators in bridges.
In life-cycle cost optimization, metaheuristic (Noureldin et al. 2021; Razavi and Gholizadeh 2021) and gradient-based (Kleingesinds and Lavan 2021) algorithms have been implemented in addition to uniform damage distribution methods (Asadi and Hajirasouliha 2020; Mousazadeh et al. 2020). In Joyner et al. (2022), a post-Pareto pruning approach was proposed to determine a manageable number of final designs in multiobjective optimization. Resilience-based performance targets have also been defined as an objective function (Joyner et al. 2022).
Life-cycle cost optimization has been used to design different types of structural systems, including steel moment frames (Fragiadakis et al. 2006b; Liu et al. 2003, 2005a; Wen and Kang 2001b), reinforced concrete frames (Esteva et al. 2002; Lagaros and Magoula 2013; Möller et al. 2015; Zou et al. 2007a), steel moment frames with fluid viscous dampers (Ahadzadeh Kolour et al. 2021; Gidaris and Taflanidis 2015; Shin and Singh 2014b), steel frames with tuned mass dampers (Matta 2018), steel frames with yielding metallic devices (Shin and Singh 2017), steel braced frames (Park et al. 2015), lead rubber base isolation systems (Mousazadeh et al. 2020), steel frames with steel panel walls (Jiang et al. 2020), and reinforced concrete frames with friction dampers and disc springs (Noureldin et al. 2021). To quantify the benefits of using expensive materials, some studies have implemented a life-cycle cost optimization methodology. Gencturk (2013) optimized the life-cycle cost of conventional reinforced concrete and reinforced engineered cementitious composite frames. Life-cycle cost assessment has also been used to assess and compare optimally designed structures (Gencturk 2013; Lagaros et al. 2010). Life-cycle cost optimization methodologies have also been implemented to design structures with in-plane irregularities (de Salles et al. 2023).
Different types of analyses have been used in life-cycle cost optimization. For this purpose, some studies have utilized conventional pushover analyses (Esteva et al. 2002; Fragiadakis et al. 2006b; Liu 2005; Liu et al. 2003, 2004; Zou et al. 2007a). Modified modal pushover analysis has also been used to capture higher mode effects in total life-cycle cost optimization (Li et al. 2012). In Mitropoulou et al. (2011), NRHAs were implemented in assessing the life-cycle cost of three-dimensional (3D) reinforced concrete buildings. Mitropoulou et al. (2011) studied the impact of analysis type, number of earthquake ground motions, and structure irregularity on life-cycle cost. The response spectrum method has been used to reduce the computational time in performing life-cycle cost optimization (Shin and Singh 2014a, b). The effectiveness of the endurance time method as the analysis engine has also been evaluated (Basim and Estekanchi 2015). The endurance time method is a single response history analysis that evaluates the behavior of the structure at continuous intensity levels by applying intensified accelerograms as loading functions.
Some studies have utilized the FEMA P-58 methodology to determine expected annual economic and social losses (Ahmadie Amiri and Estekanchi 2023; Khalilian et al. 2021; Mirfarhadi et al. 2021; Saadat et al. 2014; Varaee et al. 2021). The contributions of drift-, acceleration-, and velocity-sensitive components to losses were evaluated in Ghasemof et al. (2022a, b). A value-based seismic design methodology was proposed in Mirfarhadi et al. (2021) to minimize the total cost. The seismic consequences, including the repair cost, repair time, injury, and fatality, were evaluated.
Life-cycle cost optimization has also been used for structures subjected to wind actions (Beck et al. 2014; Wen and Kang 2001b). In some studies (Kleingesinds and Lavan 2022), the life-cycle cost of structures was considered as a performance constraint in multihazard PBDO of tuned mass dampers. Some studies (Kleingesinds and Lavan 2021, 2022) assumed that tall buildings subjected to wind loads behave linearly, which means that only nonstructural damage was considered in the life-cycle cost evaluation (Kleingesinds and Lavan 2021, 2022).
Discussion
The life-cycle cost optimization of buildings with a focus on size variables has been studied (Ghaderi and Gholizadeh 2021; Ghasemof et al. 2022a; Wen and Kang 2001a). However, the life-cycle cost topology-based optimal design of structural systems is yet to be examined.
Life-cycle cost optimization has only been applied to a few types of structural systems (Ahadzadeh Kolour et al. 2021; Möller et al. 2015; Wen and Kang 2001a). Future studies on the life-cycle cost optimization of steel braced frames, steel and reinforced concrete shear wall systems, and self-centering systems are recommended.
High-speed computing resources are needed to solve life-cycle cost optimization problems. Computational expense can be minimized using machine learning techniques, as explained in the “Approaches to Reducing Computational Time” section.
The use of drift and acceleration limits has not been consistent across different life-cycle cost assessment studies (Liu et al. 2004; Wen and Kang 2001a). Therefore, fragility functions have been utilized to calculate the life-cycle cost in the optimization framework (Jiang et al. 2020; Shin and Singh 2014b). The P-58 is suggested as the basis for determining the life-cycle cost in a consistent and reliable manner (Khalilian et al. 2021; Mirfarhadi et al. 2021; Saadat et al. 2014; Varaee et al. 2021). Research on the life-cycle cost optimization of structures under wind loading is limited. Consequently, a significant emphasis in research studies should be directed toward the life-cycle cost optimization of structural systems subjected to wind loads.
There are some limitations in the prior research studies that implemented the FEMA P-58 methodology to determine the life-cycle cost in the optimization (Ghasemof et al. 2022b). The performance metrics of the optimized structures were calculated based on a specific location and occupancy type (Ghasemof et al. 2022b). In addition, the results of these studies are dependent on the quantity and type of vulnerable contents in the structures selected by the authors.
Repair Cost Using the Park–Ang Damage Index
Deterministic Formulation
In the last two decades, the Park–Ang damage index (Park et al. 1985) has been used to determine the repair cost of structures following severe earthquakes. The global Park–Ang damage index is defined as follows:where , , and are, respectively, the maximum roof displacement, the ultimate roof displacement, and the yield roof displacement of the structure; is the yield strength; is a nonnegative strength deteriorating constant; and is the absorbed hysteretic energy.
(9)
The local Park–Ang damage index can be determined using Eq. (10) (Fattahi and Gholizadeh 2019; Gholizadeh and Fattahi 2018)where , , and are, respectively, the maximum, ultimate, and recoverable rotation of structural elements; and is the yield moment of the structural element.
(10)
In a study by Hajirasouliha et al. (2012), the coefficient of variation of the damage index in reinforced concrete frames was minimized. The frames were considered optimum if the coefficient of variation of the damage index for beams and columns was less than 0.1 (Hajirasouliha et al. 2012).
Following the calculation of the local Park–Ang damage index, the global index is calculated as follows:where is the Park–Ang-based global damage index, is the number of elements and is the energy absorbed by the th element.
(11)
(12)
The results from a study by Hajirasouliha et al. (2012) show that by using the Park–Ang index in the optimization, the damage is reduced compared to structures that are designed according to the 2009 International Building Code (IBC) (ICC 2009) (shown in Fig. 6).
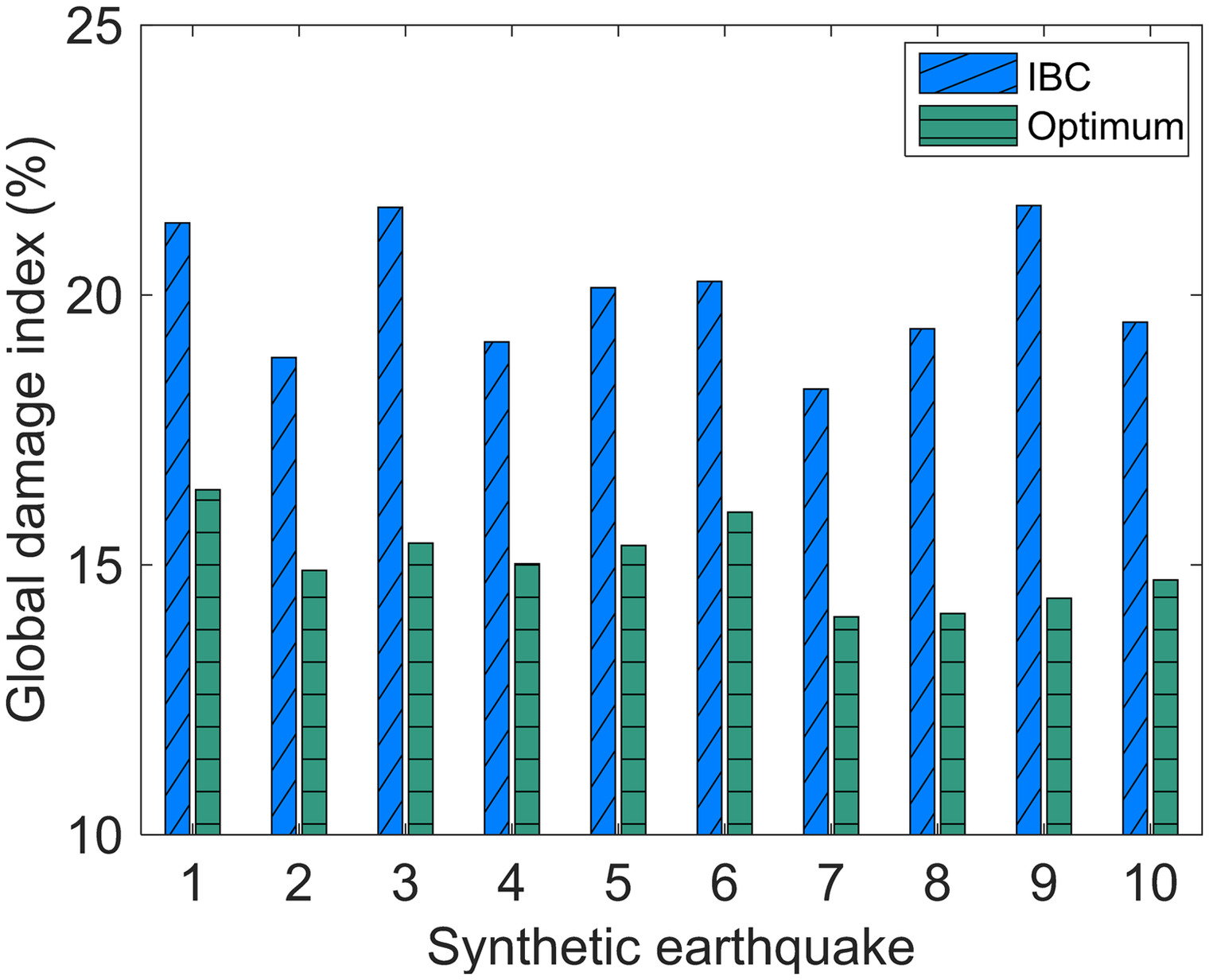
Damage states for steel moment frames based on the Park–Ang damage index are given in Table 6.
The repair cost using the Park–Ang damage index is calculated as follows:where is a factor that considers the demolition and clearing costs and is the damage index corresponding to the repairability state.
(13)
The expected repair cost at time considers a mean annual rate of earthquake occurrence, , and a discount rate, , which can be written as follows (Möller et al. 2009, 2015):
(14)
The Park–Ang damage index can be incorporated by defining a constraint in the optimization process (Fattahi and Gholizadeh 2019; Gholizadeh and Fattahi 2018). Alternatively, the expected repair cost using the Park–Ang damage index can be defined as an objective function in the optimization process (Fattahi and Gholizadeh 2019).
Probabilistic Formulation
In the repair cost optimization using the Park–Ang damage index within PBD, probabilistic constraints can also be defined to consider different sources of uncertainty. The reliability index, calculated using MCS, has been considered as a constraint in the optimization (Khatibinia et al. 2013; Yazdani et al. 2017).
Discussion
As presented in Table 6, different criteria are defined as damage states in using the Park–Ang damage index. This inconsistency in the defined damage states is a major limitation of using the Park–Ang damage index for the cost optimization of structures. Park–Ang damage indices have been implemented for the cost optimization of only reinforced concrete and steel moment frames (Gholizadeh and Fattahi 2018; Hajirasouliha et al. 2012). Park–Ang-based cost assessment is based on system-level damage, which makes it impossible to consider how enhancing the robustness of specific components can be used to reduce losses. Therefore, we recommend using the component-based FEMA P-58 methodology to assess losses.
PBDO for Retrofitting Structures
Previous Research and Current Practice
In the last two decades, PBDO has been implemented to efficiently retrofit existing structures (e.g., Altieri et al. 2018; Cha and Agrawal 2017; Dehghani et al. 2019; Idels and Lavan 2021b; Mohammadi et al. 2019). The optimal retrofit design has been studied for structures with different types of energy dissipation devices, including linear fluid viscous dampers (Lavan 2015; Lavan and Dargush 2009; Liu et al. 2005b; Sarcheshmehpour et al. 2020; Steneker et al. 2022), nonlinear fluid viscous dampers (De Domenico and Hajirasouliha 2021; Liu et al. 2005b), viscoelastic dampers (Tubaldi et al. 2016; Lavan and Dargush 2009), magnetorheological dampers (Cha et al. 2014; Cha and Agrawal 2017; Cha and Bai 2016), tuned impact dampers (Lu et al. 2018), friction dampers (Moghaddam et al. 2022; Nabid et al. 2019; Sanghai and Pawade 2021), curved dampers (Fathizadeh et al. 2020), steel slit dampers (Noureldin et al. 2022), and buckling-restrained braces (Lavan and Dargush 2009; Tu et al. 2020, 2022; Laguardia and Franchin 2022). To rehabilitate existing structures, X-braces have been implemented (Dehghani et al. 2019) with the weight and story drifts considered in the objective function. Optimization methodologies have also been used to effectively reduce the amount of fiber-reinforced polymer composites required for a given retrofit project (Zou et al. 2018, 2007b). This minimization not only lowers the overall cost but also improves the efficiency of the retrofit process. In recent years, optimization methodologies have been used to retrofit irregular structures. Gradient-based seismic design approaches have been used for the design of 3D irregular structures, incorporating multiple tuned mass dampers (Daniel and Lavan 2014) and nonlinear fluid viscous dampers (Pollini et al. 2017).
A challenge in retrofitting structures using energy dissipation devices is to find their optimal parameters and distribution in the structure (Liu et al. 2005b; Sarcheshmehpour et al. 2020). PBDO can address this challenge and minimize structural response demands (De Domenico and Hajirasouliha 2021; Lavan and Dargush 2009; Liu et al. 2005b; Noureldin et al. 2022). This procedure has been used to consider objective functions that include the device cost (Lavan 2015; Liu et al. 2005b), number of dampers (Cha et al. 2014), material cost (Tu et al. 2022), maximum story drift (Cha et al. 2014; De Domenico and Hajirasouliha 2021; Lavan and Dargush 2009; Liu et al. 2005b; Noureldin et al. 2022; Tu et al. 2022), absolute floor acceleration (Lavan and Dargush 2009; Liu et al. 2005b), response error (Lu et al. 2018), structural stiffness uniformity (Lu et al. 2018), summation of the slip loads (Nabid et al. 2019), maximum shear of the structure (Sanghai and Pawade 2021), and cumulative and maximum strain energy of the structure (Moghaddam et al. 2022). Moreover, some of the objective function variables (e.g., maximum story drift, absolute acceleration, and force in each damper) can be considered as constraints in the optimization (Lavan 2015).
A significant challenge in PBDO arises when selecting discrete design parameters, such as steel cross sections from standard charts, which results in a mixed-integer optimization problem (Idels and Lavan 2021a). To address this issue using a gradient-based algorithm, the optimization problem is converted to a continuous formulation, significantly reducing the computational time (Idels and Lavan 2021a). Then, the final design can be achieved by applying a material interpolation function (Pollini et al. 2017).
In implementing the PBDO methodology to retrofit existing structures, some studies have considered the life-cycle cost of structures as an objective function in optimization (El-Khoury et al. 2018; Gidaris and Taflanidis 2015; Jiang et al. 2020; Matta 2018). Furthermore, using FEMA P-58, an integrated structural and nonstructural retrofit optimization methodology was proposed in Steneker et al. (2020) to reduce economic loss, downtime, and the number of casualties. They proposed a median shift probability method to evaluate the effect of structural upgrades on nonstructural components (Steneker et al. 2022). Moreover, component-level fragility functions have been used to assess the repair cost of bridges with optimal mechanical properties of isolation bearings and fluid dampers (Xie and Zhang 2017, 2018).
In recent years, a few methodologies have been proposed for the resilience-based design of structures (Angeles et al. 2021; Wang et al. 2023). PBDO has also been used to retrofit and improve the seismic resilience of existing structures. Recently, a multiobjective PBDO methodology was developed to retrofit existing reinforced concrete frames (Omidian and Khaji 2022). The study considered retrofit elements made from steel, glass fiber reinforced polymer (GFRP), and carbon fiber reinforced polymer (CFRP) with different thicknesses and layers. The resilience and retrofit cost indices were considered in the objective functions. The resilience index was calculated as follows:where and represent the performance of the structure and the control time (e.g., life cycle, system lifetime).
(15)
To implement PBDO methodologies for structural retrofit, genetic (Lavan and Dargush 2009; Liu et al. 2005b), gradient-based (Lavan 2015), and uniform damage distribution optimization algorithms (De Domenico and Hajirasouliha 2021; Moghaddam et al. 2022; Mohammadi et al. 2019; Nabid et al. 2019) have been utilized. Moreover, structural response simulation in the optimization process has been implemented using linear (Lavan 2015) and nonlinear response history analysis (Cha et al. 2014), and endurance time method (Sarcheshmehpour et al. 2020). It is notable that very few studies have considered soil–structure interaction in the design optimization process (Sanghai and Pawade 2021; Sarcheshmehpour et al. 2020).
Discussion
Most of the previous research on optimal retrofit design (Grierson et al. 2006; Liu et al. 2005b; Xu et al. 2006) only considered the initial cost of the structural or energy dissipation device as the objective function. Future works should include the life-cycle cost of structural and nonstructural components in the optimization. Environmental impacts should also be considered as a performance metric in future studies on PBDO-based structural retrofit. In addition to seismic loading, wind loading should also be considered in the retrofit design process. Further investigations are needed to evaluate the implications of plan and vertical irregularities (using 3D models) in PBDO-based structural retrofits.
Approaches to Reducing Computational Time
One of the main challenges in implementing PBDO using metaheuristic algorithms is their computational expense. In the last two decades, several methods, including artificial intelligence (Yazdani et al. 2017), surrogate modeling (Gidaris and Taflanidis 2015), design of experiments (Möller et al. 2015), and simplified nonlinear structural analyses (Basim and Estekanchi 2015), have been implemented to reduce the computational time required for PBDO. Prior research using these approaches is summarized in the following subsection.
Artificial Intelligence
Some studies have implemented neural network (NN) techniques to reduce the required computational time for PBDO (Lagaros et al. 2008; Noureldin et al. 2022; Yazdani et al. 2017). Lagaros et al. (2008) implemented feed-forward NNs to predict the reliability of optimal designs using the first-order reliability method. Another study (Kaveh et al. 2012) implemented feed-forward back-propagation, cascade-forward back-propagation, radial basis function, and generalized regression methods to estimate structural responses in PBDO (Gholizadeh and Fattahi 2018). The radial basis function and generalized regression NN are two feed-forward NN layers, and the Gaussian transfer function was used as the hidden layer (Gholizadeh and Fattahi 2018). In Noureldin et al. (2022), NNs were used to predict three types of EDPs. NNs have also been utilized to generate safety margin curves for structural retrofit in determining the optimal placement of dampers (Noureldin et al. 2022).
Wavelets have also been used as activation functions in the hidden layers of NNs (Gholizadeh and Mohammadi 2017). Specifically, wavelet NNs have been used to predict structural response parameters to reduce the computational time in the optimization (Gholizadeh and Fattahi 2018; Kaveh et al. 2014). In Gholizadeh (2015), an NN was developed where the wavelet function was used as an alternative to the hyperbolic tangent sigmoid function in the hidden layers with the goal of overcoming the overfitting problem with a standard back-propagation NN. Wavelet NNs have also been implemented in reliability estimation using MCS (Gholizadeh and Aligholizadeh 2019; Gholizadeh and Mohammadi 2017).
Design of Experiments and Surrogate Modeling
The design of experiments (DoE) method has been used to construct response databases. DoE methods have been used to identify the most significant factors affecting structural response (Moradi and Burton 2018). In reliability-based design and life-cycle cost optimization, DoE has been used to generate a response database (Möller et al. 2009, 2015; Zhang and Foschi 2004). The generated database was used to train an NN to be implemented in the optimization.
Surrogate models are compact statistical representations of a mechanistic relationship. In PBDO, surrogate modeling has been implemented to approximate the relationship between input variables and output responses, resulting in a reduction in computational time (Franchini et al. 2022). In Gidaris and Taflanidis (2015), the optimization time was reduced by developing a surrogate model based on the structural response of a small number of damper configurations in a 3-story reinforced concrete building. To find the accuracy of the developed surrogate model, the life-cycle cost of the final optimal design was directly determined, in which a 3% difference was observed between the actual and estimated values. Surrogate models have also been integrated with MCS to formulate parameterized fragility functions (Moradi et al. 2018).
The response surface method has also been used for optimizing complex systems. In a study by Moradi and Burton (2018), the response surface methodology was utilized to determine the optimal design variable values with the concurrent minimization of the maximum story and residual roof drift ratios and the peak floor acceleration.
Other Methods
Other methods have also been used to decrease the PBDO runtime. Parallel computing was implemented to reduce the time associated with performing NRHAs in Fragiadakis et al. (2006a) and Park et al. (2015). Pushover analyses have been used in early iterations of the optimization process to alleviate the computational demand associated with NRHAs. Response history analyses have also been applied to only feasible designs to reduce the computational time of an optimization routine (Mokarram and Banan 2018; Razmara Shooli et al. 2019). In addition, the endurance time method (Basim and Estekanchi 2015) offers efficiency in assessing the nonlinear response of structures (Basim and Estekanchi 2015; Sarcheshmehpour et al. 2020). Furthermore, the optimization loop and reliability assessment were decoupled to reduce the overall computational time (Fathali and Vaez 2023; Spence and Gioffrè 2012). Moreover, techniques developed for general optimization purposes, including the utilization of inexact gradients (Amir 2021) and multilevel methods (Ferrari and Sigmund 2020), can be implemented to reduce computational expense.
To further reduce the computational effort, the optimization methodology can be started using one “active” ground motion record that gives the largest spectral displacements corresponding to the structure’s natural period (Lavan and Levy 2005). If the optimal design does not meet the constraints for other records within the original ensemble, additional ground motions are individually added to the “active” set until an optimum solution is achieved.
Discussion
Attempts to reduce the optimization computational time have been limited to the size optimization (Gholizadeh and Mohammadi 2017; Gidaris and Taflanidis 2015; Lagaros et al. 2008; Noureldin et al. 2022). Similar studies are needed to address the performance-based topology optimization of structures. Moreover, the approaches for reducing computational time have been integrated into PBDO only with the initial cost as the objective function. Different methods can be explored to improve the computational efficiency of the life-cycle cost optimization of structures.
Concluding Remarks
This paper systematically reviews the literature on performance-based design optimization (PBDO) of structures, including buildings and bridges under wind and seismic loading. Prior studies are summarized with an emphasis on different problem formulations. PBDO methodologies have evolved to embody several types of deterministic or probabilistic objective functions and constraints that can aid in designing cost-effective structures with predictable performance. Despite the robust body of work that has been done in this area, several unresolved issues remain. To ensure the practicality of PBDO for use in structural engineering practice, the following challenges must be addressed:
•
The current utilization of PBDO predominantly centers on the design of 2D frame structures. To broaden its applicability and relevance in real-world engineering problems, user-friendly software frameworks for PBDO that can readily be utilized by practicing engineers should be developed.
•
Most research papers have used optimization to design conventional structural systems in the PBD framework. However, a significant gap exists in implementing these methodologies for designing novel structural systems and materials, such as earthquake-resilient rocking frames and self-centering systems with shape memory alloys.
•
In PBDO studies seeking to minimize upfront costs, only the structural and nonstructural elements have been considered. Future studies should also consider the foundation, which can contribute significantly to the total upfront cost of the structure.
•
Most PBDO prior studies on new design or retrofit have addressed symmetric structural systems. The presence of torsional effects in asymmetric structural systems may have significant implications for the optimal design and should, therefore, also be considered (using 3D structural models) along with plan and vertical irregularities.
•
PBDO methodologies should also be implemented in the design of high-rise building structures.
•
Many research studies define constraints and objective functions based on the structural response at various performance levels. Collapse safety also needs to be included in the optimization of structures.
•
In life-cycle cost optimization, inconsistencies have been observed in establishing the drift and acceleration limits. The methodology presented in FEMA P-58 can be used as the basis for the life-cycle cost assessment of structures.
•
A few studies have proposed methodologies for PBDO that incorporate resilience-based objective functions. However, there is a recognized need to further advance the development of resilience-based design frameworks within the context of PBDO.
•
Many research studies have used PBDO methodologies to design structures against seismic loading. Comparatively, very few studies have addressed multihazard analysis within PBDO incorporating both wind and seismic loading.
•
To enhance the practicality of optimization methodologies, various computational expense reduction techniques have been used. These methods include artificial intelligence, design of experiments and surrogate modeling, simplified analysis methods, and computationally efficient numerical methods. However, a considerable gap exists in reducing the computational expense of performance-based life-cycle cost optimization.
•
The computational time for PBDO of structures considering size-related design variables has been effectively reduced. However, for the topology optimization of structures within the PBD context, future research is needed to apply or develop techniques that can reduce the runtime.
Data Availability Statement
Some or all data, models, or code that support the findings of this study are available from the corresponding author upon reasonable request.
Acknowledgments
This research has been supported by the Toronto Metropolitan University Faculty of Engineering and Architectural Science and the Natural Sciences and Engineering Research Council of Canada (NSERC) through Discovery Grant. The authors gratefully acknowledge the financial support.
References
Ahadzadeh Kolour, N., M. Charkhtab Basim, M. Chenaghlou, N. A. Kolour, M. C. Basim, and M. Chenaghlou. 2021. “Multi-objective optimum design of nonlinear viscous dampers in steel structures based on life cycle cost.” Structures 34 (Feb): 3776–3788. https://doi.org/10.1016/j.istruc.2021.09.100.
Ahmadie Amiri, H., and H. E. Estekanchi. 2023. “Life cycle cost-based optimization framework for seismic design and target safety quantification of dual steel buildings with buckling-restrained braces.” Earthquake Eng. Struct. Dyn. 52 (13): 4048–4081. https://doi.org/10.1002/eqe.3864.
AISC. 2016. Specification for structural steel buildings. ANSI/AISC 360-16. Chicago: AISC.
Alimoradi, A., S. Pezeshk, and C. M. Foley. 2007. “Probabilistic performance-based optimal design of steel moment-resisting frames. II: Applications.” J. Struct. Eng. 133 (6): 767–776. https://doi.org/10.1061/(ASCE)0733-9445(2007)133:6(767).
Alkhatib, F., N. Kasim, S. Qaidi, H. M. Najm, and M. M. Sabri Sabri. 2022. “Wind-resistant structural optimization of irregular tall building using CFD and improved genetic algorithm for sustainable and cost-effective design.” Front. Energy Res. 10 (Jun): 1017813. https://doi.org/10.3389/fenrg.2022.1017813.
Altieri, D., E. Tubaldi, M. De Angelis, E. Patelli, and A. DallAsta. 2018. “Reliability-based optimal design of nonlinear viscous dampers for the seismic protection of structural systems.” Bull. Earthquake Eng. 16 (2): 963–982. https://doi.org/10.1007/s10518-017-0233-4.
Amir, O. 2021. “Efficient stress-constrained topology optimization using inexact design sensitivities.” Int. J. Numer. Methods Eng. 122 (13): 3241–3272. https://doi.org/10.1002/nme.6662.
Angeles, K., D. Patsialis, A. A. Taflanidis, T. L. Kijewski-Correa, A. Buccellato, and C. Vardeman. 2021. “Advancing the design of resilient and sustainable buildings: An integrated life-cycle analysis.” J. Struct. Eng. 147 (3): 4020341. https://doi.org/10.1061/(ASCE)ST.1943-541X.0002910.
Asadi, P., and I. Hajirasouliha. 2020. “A practical methodology for optimum seismic design of RC frames for minimum damage and life-cycle cost.” Eng. Struct. 202 (Mar): 109896. https://doi.org/10.1016/j.engstruct.2019.109896.
ASCE. 2017. Seismic evaluation and retrofit of existing buildings. ASCE-41. Reston, VA: ASCE.
ATC (Applied Technology Council). 1996. Seismic evaluation and retrofit of concrete buildings. ATC-40. Redwood City, CA: ATC.
ATC (Applied Technology Council). 2003. Preliminary evaluation of methods for defining performance. ATC-58-2. Redwood City, CA: ATC.
Attary, N., V. U. Unnikrishnan, J. W. van de Lindt, D. T. Cox, and A. R. Barbosa. 2017. “Performance-based tsunami engineering methodology for risk assessment of structures.” Eng. Struct. 141 (Apr): 676–686. https://doi.org/10.1016/j.engstruct.2017.03.071.
Ayyash, A. N. A., and F. Hejazi. 2023. “Development of hybrid performance-based optimization algorithm for structures equipped with vibration damper devices.” Arch. Civ. Mech. Eng. 23 (2): 1–34. https://doi.org/10.1007/s43452-023-00665-z.
Bai, J., S. Jin, and J. Ou. 2020. “An efficient method for optimizing the seismic resistance of reinforced concrete frame structures.” Adv. Struct. Eng. 23 (4): 670–686. https://doi.org/10.1177/1369433219878856.
Basim, M. C., and H. E. Estekanchi. 2015. “Application of endurance time method in performance-based optimum design of structures.” Struct. Saf. 56 (Jun): 52–67. https://doi.org/10.1016/j.strusafe.2015.05.005.
Beck, A. T. A. T., I. A. Kougioumtzoglou, and K. R. M. M. dos Santos. 2014. “Optimal performance-based design of non-linear stochastic dynamical RC structures subject to stationary wind excitation.” Eng. Struct. 78 (Jan): 145–153. https://doi.org/10.1016/j.engstruct.2014.07.047.
Bobby, S., S. M. J. Spence, E. Bernardini, and A. Kareem. 2014. “Performance-based topology optimization for wind-excited tall buildings: A framework.” Eng. Struct. 74 (Apr): 242–255. https://doi.org/10.1016/j.engstruct.2014.05.043.
Bobby, S., S. M. J. Spence, and A. Kareem. 2016. “Data-driven performance-based topology optimization of uncertain wind-excited tall buildings.” Struct. Multidiscip. Optim. 54 (6): 1379–1402. https://doi.org/10.1007/s00158-016-1474-6.
Burton, H. V., J. Y. Lee, S. Moradi, and S. Dastmalchi. 2019. “Multi-objective performance-based design optimization of a controlled rocking steel braced frame system.” In Resilient structures and infrastructure, 243–268. Singapore: Springer.
Cha, Y. J., and J. W. Bai. 2016. “Seismic fragility estimates of a moment-resisting frame building controlled by MR dampers using performance-based design.” Eng. Struct. 116 (Jun): 192–202. https://doi.org/10.1016/j.engstruct.2016.02.055.
Cha, Y.-J. J., and A. K. Agrawal. 2017. “Seismic retrofit of MRF buildings using decentralized semi-active control for multi-target performances.” Earthquake Eng. Struct. Dyn. 46 (3): 409–424. https://doi.org/10.1002/eqe.2796.
Cha, Y.-J. J., A. K. Agrawal, B. M. Phillips, and B. F. Spencer. 2014. “Direct performance-based design with 200kN MR dampers using multi-objective cost effective optimization for steel MRFs.” Eng. Struct. 71 (Sep): 60–72. https://doi.org/10.1016/j.engstruct.2014.04.023.
Chan, C.-M. M., and X.-K. K. Zou. 2004. “Elastic and inelastic drift performance optimization for reinforced concrete buildings under earthquake loads.” Earthquake Eng. Struct. Dyn. 33 (8): 929–950. https://doi.org/10.1002/eqe.385.
Ciampoli, M., F. Petrini, and G. Augusti. 2011. “Performance-based wind engineering: Towards a general procedure.” Struct. Saf. 33 (6): 367–378. https://doi.org/10.1016/j.strusafe.2011.07.001.
Daniel, Y., and O. Lavan. 2014. “Gradient based optimal seismic retrofitting of 3D irregular buildings using multiple tuned mass dampers.” Comput. Struct. 139 (Aug): 84–97. https://doi.org/10.1016/j.compstruc.2014.03.002.
De Domenico, D., and I. Hajirasouliha. 2021. “Multi-level performance-based design optimisation of steel frames with nonlinear viscous dampers.” Bull. Earthquake Eng. 19 (12): 5015–5049. https://doi.org/10.1007/s10518-021-01152-7.
De Domenico, D., G. Ricciardi, and I. Takewaki. 2019. “Design strategies of viscous dampers for seismic protection of building structures: A review.” Soil Dyn. Earthquake Eng. 118 (Feb): 144–165. https://doi.org/10.1016/j.soildyn.2018.12.024.
Dehghani, S., A. R. Vosoughi, and M. R. Banan. 2019. “The effects of rehabilitation objectives on near optimal trade-off relation between minimum weight and maximum drift of 2D steel X-braced frames considering soil-structure interaction using a cluster-based NSGA II.” Struct. Multidiscip. Optim. 59 (5): 1703–1722. https://doi.org/10.1007/s00158-018-2153-6.
Del Gobbo, G. M., M. S. Williams, and A. Blakeborough. 2018. “Seismic performance assessment of Eurocode 8-compliant concentric braced frame buildings using FEMA P-58.” Eng. Struct. 155 (Apr): 192–208. https://doi.org/10.1016/j.engstruct.2017.11.016.
Deng, T., J. Fu, Q. Zheng, J. Wu, and Y. Pi. 2019. “Performance-based wind-resistant optimization design for tall building structures.” J. Struct. Eng. 145 (10): 4019103. https://doi.org/10.1061/(ASCE)ST.1943-541X.0002383.
de Salles, H. B., L. F. F. Miguel, M. S. Lenzi, R. H. Lopez, and A. T. Beck. 2023. “A Fast Frequency Sweep approach for performance-based optimization of earthquake-resistant irregular large-scale buildings.” Eng. Struct. 285 (Mar): 116094. https://doi.org/10.1016/j.engstruct.2023.116094.
Dong, G., I. Hajirasouliha, K. Pilakoutas, and P. Asadi. 2023. “Multi-level performance-based seismic design optimisation of RC frames.” Eng. Struct. 293 (Jan): 116591. https://doi.org/10.1016/j.engstruct.2023.116591.
Ebadijalal, M., and M. Shahrouzi. 2022. “Evaluating ASCE 41-17 performance-based provisions on optimally designed steel moment frames.” Struct. Des. Tall Special Build. 31 (17): e1977. https://doi.org/10.1002/tal.1977.
Eftekhar, B., O. Rezaifar, and M. S. Karimi. 2022. “A new hybrid meta-heuristic algorithm for optimum performance-based seismic designs of moment-resisting frames.” Eng. Optim. 54 (6): 1023–1043. https://doi.org/10.1080/0305215X.2021.1907574.
Eguchi, R. T., J. D. Goltz, C. E. Taylor, S. E. Chang, P. J. Flores, L. A. Johnson, H. A. Seligson, and N. C. Blais. 1998. “Direct economic losses in the Northridge earthquake: A three-year post-event perspective.” Earthquake Spectra 14 (2): 245–264. https://doi.org/10.1193/1.1585998.
El-Khoury, O., A. Shafieezadeh, and E. Fereshtehnejad. 2018. “A risk-based life cycle cost strategy for optimal design and evaluation of control methods for nonlinear structures.” Earthquake Eng. Struct. Dyn. 47 (11): 2297–2314. https://doi.org/10.1002/eqe.3069.
Esteva, L., O. Díaz-López, J. García-Pérez, G. Sierra, and E. Ismael. 2002. “Life-cycle optimization in the establishment of performance-acceptance parameters for seismic design.” Struct. Saf. 24 (2–4): 187–204. https://doi.org/10.1016/S0167-4730(02)00024-3.
Fathali, M. A., and S. R. Hoseini Vaez. 2020. “Optimum performance-based design of eccentrically braced frames.” Eng. Struct. 202 (Mar): 109857. https://doi.org/10.1016/j.engstruct.2019.109857.
Fathali, M. A., and S. R. H. Vaez. 2023. “A decoupled double-loop method with the adaptive allowable limits for probabilistic performance-based design optimization.” Eng. Struct. 279 (Apr): 115634. https://doi.org/10.1016/j.engstruct.2023.115634.
Fathizadeh, S. F., S. Dehghani, T. Y. Yang, E. Noroozinejad Farsangi, A. R. Vosoughi, I. Hajirasouliha, I. Takewaki, C. Málaga-Chuquitaype, and H. Varum. 2020. “Trade-off Pareto optimum design of an innovative curved damper truss moment frame considering structural and non-structural objectives.” Structures 28 (Dec): 1338–1353. https://doi.org/10.1016/j.istruc.2020.09.060.
Fathizadeh, S. F., A. R. Vosoughi, and M. R. Banan. 2021. “Considering soil–structure interaction effects on performance-based design optimization of moment-resisting steel frames by an engineered cluster-based genetic algorithm.” Eng. Optim. 53 (3): 440–460. https://doi.org/10.1080/0305215X.2020.1739278.
Fattahi, F., and S. Gholizadeh. 2019. “Seismic fragility assessment of optimally designed steel moment frames.” Eng. Struct. 179 (Aug): 37–51. https://doi.org/10.1016/j.engstruct.2018.10.075.
FEMA. 2000a. Prestandard and commentary for the seismic rehabilitation of buildings. FEMA-356. Washington, DC: FEMA.
FEMA. 2000b. Recommended seismic design criteria for new moment-resisting steel frame structures. FEMA-350. Washington, DC: FEMA.
FEMA. 2012. Seismic performance assessment of buildings volume 1-methodology. FEMA-P58-1. Washington, DC: FEMA.
FEMA. 2013. Multi-hazard loss estimation methodology, earthquake model, Hazus-MH 2.1, technical manual. Washington, DC: FEMA.
Ferrari, F., and O. Sigmund. 2020. “Towards solving large-scale topology optimization problems with buckling constraints at the cost of linear analyses.” Comput. Methods Appl. Mech. Eng. 363 (Apr): 112911. https://doi.org/10.1016/j.cma.2020.112911.
Foley, C. M., S. Pezeshk, and A. Alimoradi. 2007. “Probabilistic performance-based optimal design of steel moment-resisting frames. I: Formulation.” J. Struct. Eng. 133 (6): 757–766. https://doi.org/10.1061/(ASCE)0733-9445(2007)133:6(757).
Fragiadakis, M., and N. D. Lagaros. 2011. “An overview to structural seismic design optimisation frameworks.” Comput. Struct. 89 (11–12): 1155–1165. https://doi.org/10.1016/j.compstruc.2010.10.021.
Fragiadakis, M., N. D. Lagaros, and M. Papadrakakis. 2006a. “Performance-based earthquake engineering using structural optimisation tools.” Int. J. Reliab. Saf. 1 (1–2): 59–76. https://doi.org/10.1504/IJRS.2006.010690.
Fragiadakis, M., N. D. Lagaros, and M. Papadrakakis. 2006b. “Performance-based multiobjective optimum design of steel structures considering life-cycle cost.” Struct. Multidiscip. Optim. 32 (1): 1–11. https://doi.org/10.1007/s00158-006-0009-y.
Fragiadakis, M., and M. Papadrakakis. 2008. “Performance-based optimum seismic design of reinforced concrete structures.” Earthquake Eng. Struct. Dyn. 37 (6): 825–844. https://doi.org/10.1002/eqe.786.
Franchin, P., F. Petrini, and F. Mollaioli. 2018. “Improved risk-targeted performance-based seismic design of reinforced concrete frame structures.” Earthquake Eng. Struct. Dyn. 47 (1): 49–67. https://doi.org/10.1002/eqe.2936.
Franchini, A., W. Sebastian, and D. D’Ayala. 2022. “Surrogate-based fragility analysis and probabilistic optimisation of cable-stayed bridges subject to seismic loads.” Eng. Struct. 256 (Jun): 113949. https://doi.org/10.1016/j.engstruct.2022.113949.
Fu, J., Q. Zheng, Y. Huang, J. Wu, Y. Pi, and Q. Liu. 2018. “Design optimization on high-rise buildings considering occupant comfort reliability and joint distribution of wind speed and direction.” Eng. Struct. 156 (Oct): 460–471. https://doi.org/10.1016/j.engstruct.2017.11.041.
Ganzerli, S., C. P. Pantelides, and L. D. Reaveley. 2000. “Performance-based design using structural optimization.” Earthquake Eng. Struct. Dyn. 29 (11): 1677–1690. https://doi.org/10.1002/1096-9845(200011)29:11%3C1677::AID-EQE986%3E3.0.CO;2-N.
Gencturk, B. 2013. “Life-cycle cost assessment of RC and ECC frames using structural optimization.” Earthquake Eng. Struct. Dyn. 42 (1): 61–79. https://doi.org/10.1002/eqe.2193.
Ghaderi, M., and S. Gholizadeh. 2021. “Mainshock–aftershock low-cycle fatigue damage evaluation of performance-based optimally designed steel moment frames.” Eng. Struct. 237 (Nov): 112207. https://doi.org/10.1016/j.engstruct.2021.112207.
Ghasemof, A., M. Mirtaheri, and R. K. Mohammadi. 2022a. “Effects of demand parameters in the performance-based multi-objective optimum design of steel moment frame buildings.” Soil Dyn. Earthquake Eng. 153 (Aug): 107075. https://doi.org/10.1016/j.soildyn.2021.107075.
Ghasemof, A., M. Mirtaheri, and R. K. Mohammadi. 2022b. “Multi-objective optimization for probabilistic performance-based design of buildings using FEMA P-58 methodology.” Eng. Struct. 254 (Sep): 113856. https://doi.org/10.1016/j.engstruct.2022.113856.
Ghasemof, A., M. Mirtaheri, R. K. Mohammadi, and M. R. Mashayekhi. 2021. “Multi-objective optimal design of steel MRF buildings based on life-cycle cost using a swift algorithm.” Structures 34 (Dec): 4041–4059. https://doi.org/10.1016/j.istruc.2021.09.088.
Ghobarah, A. 2001. “Performance-based design in earthquake engineering: State of development.” Eng. Struct. 23 (8): 878–884. https://doi.org/10.1016/S0141-0296(01)00036-0.
Gholizadeh, S. 2015. “Performance-based optimum seismic design of steel structures by a modified firefly algorithm and a new neural network.” Adv. Eng. Software 81 (C): 50–65. https://doi.org/10.1016/j.advengsoft.2014.11.003.
Gholizadeh, S., and V. Aligholizadeh. 2019. “Reliability-based optimum seismic design of RC frames by a metamodel and metaheuristics.” Struct. Des. Tall Special Build. 28 (1): e1552. https://doi.org/10.1002/tal.1552.
Gholizadeh, S., and M. Ebadijalal. 2018. “Performance based discrete topology optimization of steel braced frames by a new metaheuristic.” Adv. Eng. Software 123 (May): 77–92. https://doi.org/10.1016/j.advengsoft.2018.06.002.
Gholizadeh, S., and F. Fattahi. 2018. “Damage-controlled performance-based design optimization of steel moment frames.” Struct. Des. Tall Special Build. 27 (14): e1498. https://doi.org/10.1002/tal.1498.
Gholizadeh, S., O. Hasançebi, H. Eser, and O. Koçkaya. 2022a. “Seismic collapse safety based optimization of steel moment-resisting frames.” Structures 45 (Feb): 329–342. https://doi.org/10.1016/j.istruc.2022.09.034.
Gholizadeh, S., A. Hassanzadeh, A. Milany, and H. F. Ghatte. 2022b. “On the seismic collapse capacity of optimally designed steel braced frames.” Eng. Comput. 38 (2): 985–997. https://doi.org/10.1007/s00366-020-01096-7.
Gholizadeh, S., A. Milany, and O. Hasançebi. 2023. “Seismic optimization and performance assessment of special steel moment-resisting frames considering nonlinear soil-structure interaction.” Steel Compos. Struct. 47 (3): 339–353. https://doi.org/10.12989/scs.2023.47.3.339.
Gholizadeh, S., and R. Moghadas. 2014. “Performance-based optimum design of steel frames by an improved quantum particle swarm optimization.” Adv. Struct. Eng. 17 (2): 143–156. https://doi.org/10.1260/1369-4332.17.2.143.
Gholizadeh, S., and M. Mohammadi. 2017. “Reliability-based seismic optimization of steel frames by metaheuristics and neural networks.” ASCE-ASME J. Risk Uncertainty Eng. Syst. Part A: Civ. Eng. 3 (1): 4016013. https://doi.org/10.1061/ajrua6.0000892.
Gholizadeh, S., and H. Poorhoseini. 2016. “Seismic layout optimization of steel braced frames by an improved dolphin echolocation algorithm.” Struct. Multidiscip. Optim. 54 (4): 1011–1029. https://doi.org/10.1007/s00158-016-1461-y.
Gidaris, I., and A. A. Taflanidis. 2015. “Performance assessment and optimization of fluid viscous dampers through life-cycle cost criteria and comparison to alternative design approaches.” Bull. Earthquake Eng. 13 (4): 1003–1028. https://doi.org/10.1007/s10518-014-9646-5.
Gkimprixis, A., E. Tubaldi, and J. Douglas. 2019. “Comparison of methods to develop risk-targeted seismic design maps.” Bull. Earthquake Eng. 17 (7): 3727–3752. https://doi.org/10.1007/s10518-019-00629-w.
Gkimprixis, A., E. Tubaldi, and J. Douglas. 2020. “Evaluating alternative approaches for the seismic design of structures.” Bull. Earthquake Eng. 18 (9): 4331–4361. https://doi.org/10.1007/s10518-020-00858-4.
Goda, K., and H. P. Hong. 2006. “Optimal seismic design considering risk attitude, societal tolerable risk level, and life quality criterion.” J. Struct. Eng. 132 (12): 2027–2035. https://doi.org/10.1061/(ASCE)0733-9445(2006)132:12(2027).
Grierson, D. E., Y. Gong, and L. E. I. Xu. 2006. “Optimal performance-based seismic design using modal pushover analysis.” J. Earthquake Eng. 10 (1): 73–96. https://doi.org/10.1142/S1363246906002335.
Hajirasouliha, I., P. Asadi, and K. Pilakoutas. 2012. “An efficient performance-based seismic design method for reinforced concrete frames.” Earthquake Eng. Struct. Dyn. 41 (4): 663–679. https://doi.org/10.1002/eqe.1150.
Hassanzadeh, A., and S. Gholizadeh. 2019. “Collapse-performance-aided design optimization of steel concentrically braced frames.” Eng. Struct. 197 (Feb): 109411. https://doi.org/10.1016/j.engstruct.2019.109411.
Hassanzadeh, A., and S. Moradi. 2022. “Topology optimization and seismic collapse assessment of shape memory alloy (SMA)-braced frames: Effectiveness of Fe-based SMAs.” Front. Struct. Civ. Eng. 16 (3): 281–301. https://doi.org/10.1007/s11709-022-0807-3.
Hassanzadeh, A., S. Moradi, and H. V. Burton. 2024. “Performance-based optimum seismic design of self-centering steel moment frames with SMA-based connections.” Earthquake Eng. Struct. Dyn. 53 (5): 1905–1929. https://doi.org/10.1002/eqe.4098.
Hoseini Vaez, S. R., A. Asaad Samani, and M. A. Fathali. 2022. “Optimum performance-based design of unsymmetrical 2D steel moment frame.” Soft Comput. 26 (12): 5637–5659. https://doi.org/10.1007/s00500-022-06927-x.
Huang, M. F., C. M. Chan, and W. J. Lou. 2012. “Optimal performance-based design of wind sensitive tall buildings considering uncertainties.” Comput. Struct. 98–99 (Jun): 7–16. https://doi.org/10.1016/j.compstruc.2012.01.012.
Huang, M. F., Q. Li, C. M. Chan, W. J. Lou, K. C. S. S. Kwok, and G. Li. 2015. “Performance-based design optimization of tall concrete framed structures subject to wind excitations.” J. Wind Eng. Ind. Aerodyn. 139 (Sep): 70–81. https://doi.org/10.1016/j.jweia.2015.01.005.
Hutt, C. M., T. Rossetto, and G. G. Deierlein. 2019. “Comparative risk-based seismic assessment of 1970s vs modern tall steel moment frames.” J. Constr. Steel Res. 159 (Aug): 598–610. https://doi.org/10.1016/j.jcsr.2019.05.012.
ICC (International Code Council). 2009. 2009 international building code. Country Club Hills, IL: ICC.
Idels, O., and O. Lavan. 2020. “Performance based formal optimized seismic design of steel moment resisting frames.” Comput. Struct. 235 (Jan): 106269. https://doi.org/10.1016/j.compstruc.2020.106269.
Idels, O., and O. Lavan. 2021a. “Optimization-based seismic design of steel moment-resisting frames with nonlinear viscous dampers.” Struct. Control Health Monit. 28 (1): e2655. https://doi.org/10.1002/stc.2655.
Idels, O., and O. Lavan. 2021b. “Performance-based seismic retrofitting of frame structures using negative stiffness devices and fluid viscous dampers via optimization.” Earthquake Eng. Struct. Dyn. 50 (12): 3116–3137. https://doi.org/10.1002/eqe.3502.
Idels, O., and O. Lavan. 2023. “Optimization-based seismic design of irregular self-centering moment resisting frames with ED bars or fluid viscous dampers.” Struct. Multidiscip. Optim. 66 (8): 192. https://doi.org/10.1007/s00158-023-03641-6.
Iervolino, I. 2023. “Risk-targeted seismic design: Prospects, applications, and open issues, for the next generation of building codes.” Earthquake Eng. Struct. Dyn. 52 (13): 3919–3921. https://doi.org/10.1002/eqe.3999.
Javadinasab Hormozabad, S., and M. Gutierrez Soto. 2021. “Performance-based control co-design of building structures with controlled rocking steel braced frames via neural dynamic model.” Struct. Multidiscip. Optim. 64 (3): 1111–1125. https://doi.org/10.1007/s00158-021-02902-6.
Jiang, L. L., L. L. Jiang, Y. Hu, J. Ye, and H. Zheng. 2020. “Seismic life-cycle cost assessment of steel frames equipped with steel panel walls.” Eng. Struct. 211 (Sep): 110399. https://doi.org/10.1016/j.engstruct.2020.110399.
Joyner, M. D., B. Puentes, C. Gardner, S. Steinberg, and M. Sasani. 2022. “Multiobjective optimization of building seismic design for resilience.” J. Struct. Eng. 148 (4): 4022006. https://doi.org/10.1061/(ASCE)ST.1943-541X.0003286.
Karimi, F., and S. R. Hoseini Vaez. 2019. “Two-stage optimal seismic design of steel moment frames using the LRFD-PBD method.” J. Constr. Steel Res. 155 (Mar): 77–89. https://doi.org/10.1016/j.jcsr.2018.12.023.
Kaveh, A., M. Fahimi-Farzam, and M. Kalateh-Ahani. 2015. “Optimum design of steel frame structures considering construction cost and seismic damage.” Smart Struct. Syst. 16 (1): 1–26. https://doi.org/10.12989/sss.2015.16.1.001.
Kaveh, A., B. Farahmand Azar, A. Hadidi, F. Rezazadeh Sorochi, and S. Talatahari. 2010. “Performance-based seismic design of steel frames using ant colony optimization.” J. Constr. Steel Res. 66 (4): 566–574. https://doi.org/10.1016/j.jcsr.2009.11.006.
Kaveh, A., M. Kalateh-Ahani, and M. Fahimi-Farzam. 2014. “Life-cycle cost optimization of steel moment-frame structures: Performance-based seismic design approach.” Earthquake Struct. 7 (3): 271–294. https://doi.org/10.12989/eas.2014.7.3.271.
Kaveh, A., K. Laknejadi, and B. Alinejad. 2012. “Performance-based multi-objective optimization of large steel structures.” Acta Mech. 223 (2): 355–369. https://doi.org/10.1007/s00707-011-0564-1.
Kaveh, A., R. Mahdipour Moghanni, and S. M. Javadi. 2022. “Chaotic optimization algorithm for performance-based optimization design of composite moment frames.” Eng. Comput. 38 (3): 2729–2741. https://doi.org/10.1007/s00366-020-01244-z.
Kaveh, A., and A. Nasrollahi. 2014. “Performance-based seismic design of steel frames utilizing charged system search optimization.” Appl. Soft Comput. 22 (Jun): 213–221. https://doi.org/10.1016/j.asoc.2014.05.012.
Khalilian, M., H. Shakib, and M. C. Basim. 2021. “On the optimal performance-based seismic design objective for steel moment resisting frames based on life cycle cost.” J. Build. Eng. 44 (Sep): 103091. https://doi.org/10.1016/j.jobe.2021.103091.
Khatibinia, M., E. Salajegheh, J. Salajegheh, and M. J. Fadaee. 2013. “Reliability-based design optimization of reinforced concrete structures including soil-structure interaction using a discrete gravitational search algorithm and a proposed metamodel.” Eng. Optim. 45 (10): 1147–1165. https://doi.org/10.1080/0305215X.2012.725051.
Kleingesinds, S., and O. Lavan. 2021. “Gradient-based multi-hazard optimization of MTMDs for tall buildings.” Comput. Struct. 249 (Apr): 106503. https://doi.org/10.1016/j.compstruc.2021.106503.
Kleingesinds, S., and O. Lavan. 2022. “Bi-tuned semi-active TMDs: Multi-hazard design for tall buildings using gradient-based optimization.” Struct. Control Heal. Monit. 29 (3): e2901. https://doi.org/10.1002/stc.2901.
Krogh, C., M. H. Jungersen, E. Lund, and E. Lindgaard. 2017. “Gradient-based selection of cross sections: A novel approach for optimal frame structure design.” Struct. Multidiscip. Optim. 56 (5): 959–972. https://doi.org/10.1007/s00158-017-1794-1.
Lagaros, N. D. 2014. “A general purpose real-world structural design optimization computing platform.” Struct. Multidiscip. Optim. 49 (6): 1047–1066. https://doi.org/10.1007/s00158-013-1027-1.
Lagaros, N. D., and M. Fragiadakis. 2007. “Robust performance-based design optimization of steel moment resisting frames.” J. Earthquake Eng. 11 (5): 752–772. https://doi.org/10.1080/13632460601087229.
Lagaros, N. D., and M. Fragiadakis. 2011. “Evaluation of ASCE-41, ATC-40 and N2 static pushover methods based on optimally designed buildings.” Soil Dyn. Earthquake Eng. 31 (1): 77–90. https://doi.org/10.1016/j.soildyn.2010.08.007.
Lagaros, N. D., M. Fragiadakis, M. Papadrakakis, and Y. Tsompanakis. 2006. “Structural optimization: A tool for evaluating seismic design procedures.” Eng. Struct. 28 (12): 1623–1633. https://doi.org/10.1016/j.engstruct.2006.02.014.
Lagaros, N. D., A. T. Garavelas, and M. Papadrakakis. 2008. “Innovative seismic design optimization with reliability constraints.” Comput. Methods Appl. Mech. Eng. 198 (1): 28–41. https://doi.org/10.1016/j.cma.2007.12.025.
Lagaros, N. D., and E. Magoula. 2013. “Life-cycle cost assessment of mid-rise and high-rise steel and steel-reinforced concrete composite minimum cost building designs.” Struct. Des. Tall Special Build. 22 (12): 954–974. https://doi.org/10.1002/tal.752.
Lagaros, N. D., I. A. Naziris, and M. Papadrakakis. 2010. “The influence of masonry infill walls in the framework of the performance-based design.” J. Earthquake Eng. 14 (1): 57–79. https://doi.org/10.1080/13632460902988976.
Lagaros, N. D., and M. Papadrakakis. 2007. “Seismic design of RC structures: A critical assessment in the framework of multi-objective optimization.” Earthquake Eng. Struct. Dyn. 36 (12): 1623–1639. https://doi.org/10.1002/eqe.707.
Lagaros, N. D., M. Papadrakakis, and G. Kokossalakis. 2002. “Structural optimization using evolutionary algorithms.” Comput. Struct. 80 (7–8): 571–589. https://doi.org/10.1016/S0045-7949(02)00027-5.
Laguardia, R., and P. Franchin. 2022. “Risk-based optimization of bracing systems for seismic retrofitting of RC buildings.” J. Struct. Eng. 148 (6): 4022049. https://doi.org/10.1061/(ASCE)ST.1943-541X.0003335.
Lavan, O. 2015. “Optimal design of viscous dampers and their supporting members for the seismic retrofitting of 3D irregular frame structures.” J. Struct. Eng. 141 (11): 04015026. https://doi.org/10.1061/(ASCE)ST.1943-541X.0001261.
Lavan, O., and G. F. Dargush. 2009. “Multi-objective evolutionary seismic design with passive energy dissipation systems.” J. Earthquake Eng. 13 (6): 758–790. https://doi.org/10.1080/13632460802598545.
Lavan, O., and R. Levy. 2005. “Optimal design of supplemental viscous dampers for irregular shear-frames in the presence of yielding.” Earthquake Eng. Struct. Dyn. 34 (8): 889–907. https://doi.org/10.1002/eqe.458.
Leon, R. T., R. DesRoches, J. Ocel, and G. Hess. 2001. “Innovative beam column connections using shape memory alloys.” In Proc., Smart Structures and Materials 2001: Smart Systems for Bridges, Structures, and Highways, 227–237. Bellingham, WA: SPIE.
Levy, R., and O. Lavan. 2006. “Fully stressed design of passive controllers in framed structures for seismic loadings.” Struct. Multidiscip. Optim. 32 (6): 485–498. https://doi.org/10.1007/s00158-005-0558-5.
Li, G., Y. Jiang, and D. Yang. 2012. “Modified-modal-pushover-based seismic optimum design for steel structures considering life-cycle cost.” Struct. Multidiscip. Optim. 45 (6): 861–874. https://doi.org/10.1007/s00158-011-0740-x.
Li, Y., and J. P. Conte. 2018. “Probabilistic performance-based optimum design of seismic isolation for a California high-speed rail prototype bridge.” Earthquake Eng. Struct. Dyn. 47 (2): 497–514. https://doi.org/10.1002/eqe.2976.
Liang, Q. Q. 2007. “Performance-based optimization: A review.” Adv. Struct. Eng. 10 (6): 739–753. https://doi.org/10.1260/136943307783571418.
Liu, M. 2005. “Seismic design of steel moment-resisting frame structures using multiobjective optimization.” Earthquake Spectra 21 (2): 389–414. https://doi.org/10.1193/1.1902952.
Liu, M., S. A. Burns, and Y. K. Wen. 2003. “Optimal seismic design of steel frame buildings based on life cycle cost considerations.” Earthquake Eng. Struct. Dyn. 32 (9): 1313–1332. https://doi.org/10.1002/eqe.273.
Liu, M., S. A. Burns, and Y. K. Wen. 2005a. “Multiobjective optimization for performance-based seismic design of steel moment frame structures.” Earthquake Eng. Struct. Dyn. 34 (3): 289–306. https://doi.org/10.1002/eqe.426.
Liu, M., Y. K. Wen, and S. A. Burns. 2004. “Life cycle cost oriented seismic design optimization of steel moment frame structures with risk-taking preference.” Eng. Struct. 26 (10): 1407–1421. https://doi.org/10.1016/j.engstruct.2004.05.015.
Liu, W., M. Tong, and G. C. Lee. 2005b. “Optimization methodology for damper configuration based on building performance indices.” J. Struct. Eng. 131 (11): 1746–1756. https://doi.org/10.1061/(ASCE)0733-9445(2005)131:11(1746).
Liu, Z., S. Atamturktur, and C. H. Juang. 2013. “Performance based robust design optimization of steel moment resisting frames.” J. Constr. Steel Res. 89 (Apr): 165–174. https://doi.org/10.1016/j.jcsr.2013.07.011.
Lu, Z., K. Li, Y. Ouyang, and J. Shan. 2018. “Performance-based optimal design of tuned impact damper for seismically excited nonlinear building.” Eng. Struct. 160 (Sep): 314–327. https://doi.org/10.1016/j.engstruct.2018.01.042.
Marzok, A., and O. Lavan. 2022. “Topology optimization of multiple-rocking concentrically braced frames subjected to earthquakes.” Struct. Multidiscip. Optim. 65 (4): 1–21. https://doi.org/10.1007/s00158-022-03192-2.
Marzok, A., and O. Lavan. 2023a. “Optimal seismic design of controlled coupled multiple rocking systems with viscous dampers in irregular buildings.” J. Struct. Eng. 149 (2): 4022232. https://doi.org/10.1061/JSENDH.STENG-11651.
Marzok, A., and O. Lavan. 2023b. “Optimal seismic design of multiple-rocking steel concentrically-braced frames in 3D irregular buildings.” Comput. Struct. 280 (May): 107001. https://doi.org/10.1016/j.compstruc.2023.107001.
Matta, E. 2018. “Lifecycle cost optimization of tuned mass dampers for the seismic improvement of inelastic structures.” Earthquake Eng. Struct. Dyn. 47 (3): 714–737. https://doi.org/10.1002/eqe.2987.
Mayes, R., N. Wetzel, B. Weaver, K. Tam, W. Parker, A. Brown, and D. Pietra. 2013. “Performance based design of buildings to assess damage and downtime and implement a rating system.” Bull. N. Z. Soc. Earthquake Eng. 46 (1): 40–55. https://doi.org/10.5459/bnzsee.46.1.40-55.
Mergos, P. E. 2017. “Optimum seismic design of reinforced concrete frames according to Eurocode 8 and fib Model Code 2010.” Earthquake Eng. Struct. Dyn. 46 (7): 1181–1201. https://doi.org/10.1002/eqe.2851.
Mergos, P. E. 2018. “Efficient optimum seismic design of reinforced concrete frames with nonlinear structural analysis procedures.” Struct. Multidiscip. Optim. 58 (6): 2565–2581. https://doi.org/10.1007/s00158-018-2036-x.
Mirfarhadi, S. A., H. E. Estekanchi, and M. Sarcheshmehpour. 2021. “On optimal proportions of structural member cross-sections to achieve best seismic performance using value based seismic design approach.” Eng. Struct. 231 (May): 111751. https://doi.org/10.1016/j.engstruct.2020.111751.
Mitropoulou, C. C., N. D. Lagaros, and M. Papadrakakis. 2011. “Life-cycle cost assessment of optimally designed reinforced concrete buildings under seismic actions.” Reliab. Eng. Syst. Saf. 96 (10): 1311–1331. https://doi.org/10.1016/j.ress.2011.04.002.
Moghaddam, H., F. Afzalinia, and I. Hajirasouliha. 2022. “Optimal distribution of friction dampers to improve the seismic performance of steel moment resisting frames.” Structures 37 (Jun): 624–644. https://doi.org/10.1016/j.istruc.2022.01.007.
Mohammadi, R. K., M. R. Garoosi, and I. Hajirasouliha. 2019. “Practical method for optimal rehabilitation of steel frame buildings using buckling restrained brace dampers.” Soil Dyn. Earthquake Eng. 123 (Mar): 242–251. https://doi.org/10.1016/j.soildyn.2019.04.025.
Mokarram, V., and M. R. Banan. 2018. “An improved multi-objective optimization approach for performance-based design of structures using nonlinear time-history analyses.” Appl. Soft Comput. J. 73 (Jun): 647–665. https://doi.org/10.1016/j.asoc.2018.08.048.
Möller, O., R. O. Foschi, J. P. Ascheri, M. Rubinstein, and S. Grossman. 2015. “Optimization for performance-based design under seismic demands, including social costs.” Earthquake Eng. Eng. Vib. 14 (2): 315–328. https://doi.org/10.1007/s11803-015-0025-2.
Möller, O., R. O. Foschi, L. M. Quiroz, and M. Rubinstein. 2009. “Structural optimization for performance-based design in earthquake engineering: Applications of neural networks.” Struct. Saf. 31 (6): 490–499. https://doi.org/10.1016/j.strusafe.2009.06.007.
Moradi, S., and H. V. Burton. 2018. “Response surface analysis and optimization of controlled rocking steel braced frames.” Bull. Earthquake Eng. 16 (10): 4861–4892. https://doi.org/10.1007/s10518-018-0373-1.
Moradi, S., H. V. Burton, and I. Kumar. 2018. “Parameterized fragility functions for controlled rocking steel braced frames.” Eng. Struct. 176 (Apr): 254–264. https://doi.org/10.1016/j.engstruct.2018.09.001.
Mousazadeh, M., F. Pourreza, M. C. Basim, and M. R. Chenaghlou. 2020. “An efficient approach for LCC-based optimum design of lead-rubber base isolation system via FFD and analysis of variance (ANOVA).” Bull. Earthquake Eng. 18 (4): 1805–1827. https://doi.org/10.1007/s10518-019-00754-6.
Nabid, N., I. Hajirasouliha, and M. Petkovski. 2019. “Adaptive low computational cost optimisation method for performance-based seismic design of friction dampers.” Eng. Struct. 198 (Aug): 109549. https://doi.org/10.1016/j.engstruct.2019.109549.
Noureldin, M., A. Ali, S. Memon, and J. Kim. 2022. “Fragility-based framework for optimal damper placement in low-rise moment-frame buildings using machine learning and genetic algorithm.” J. Build. Eng. 54 (Jun): 104641. https://doi.org/10.1016/j.jobe.2022.104641.
Noureldin, M., S. A. Memon, M. Gharagoz, and J. Kim. 2021. “Performance-based seismic retrofit of RC structures using concentric braced frames equipped with friction dampers and disc springs.” Eng. Struct. 243 (Dec): 112555. https://doi.org/10.1016/j.engstruct.2021.112555.
Ocel, J., M. Asce, R. Desroches, R. T. Leon, W. Gregory Hess, R. Krumme, Jack, R. Hayes, and S. Sweeney. 2004. “Steel beam-column connections using shape memory alloys.” J. Struct. Eng. 130 (5): 732–740. https://doi.org/10.1061/(ASCE)0733-9445(2004)130:5(732).
Ohsaki, M., T. Kinoshita, and P. Pan. 2007. “Multiobjective heuristic approaches to seismic design of steel frames with standard sections.” Earthquake Eng. Struct. Dyn. 36 (11): 1481–1495. https://doi.org/10.1002/eqe.690.
Omidian, P., and N. Khaji. 2022. “A multi-objective optimization framework for seismic resilience enhancement of typical existing RC buildings.” J. Build. Eng. 52 (Apr): 104361. https://doi.org/10.1016/j.jobe.2022.104361.
Pan, P., M. Ohsaki, and T. Kinoshita. 2007. “Constraint approach to performance-based design of steel moment-resisting frames.” Eng. Struct. 29 (2): 186–194. https://doi.org/10.1016/j.engstruct.2006.04.009.
Park, H. S., J. W. Hwang, and B. K. Oh. 2018. “Integrated analysis model for assessing CO2 emissions, seismic performance, and costs of buildings through performance-based optimal seismic design with sustainability.” Energy Build. 158 (Jun): 761–775. https://doi.org/10.1016/j.enbuild.2017.10.070.
Park, K., B. K. Oh, H. S. Park, and S. W. Choi. 2015. “GA-based multi-objective optimization for retrofit design on a multi-core PC cluster.” Comput. Civ. Infrastruct. Eng. 30 (12): 965–980. https://doi.org/10.1111/mice.12176.
Park, Y.-J., A. H. Ang, and Y. K. Wen. 1985. “Seismic damage analysis of reinforced concrete buildings.” J. Struct. Eng. 111 (4): 740–757. https://doi.org/10.1061/(ASCE)0733-9445(1985)111:4(740).
Pollini, N., O. Lavan, and O. Amir. 2017. “Minimum-cost optimization of nonlinear fluid viscous dampers and their supporting members for seismic retrofitting.” Earthquake Eng. Struct. Dyn. 46 (12): 1941–1961. https://doi.org/10.1002/eqe.2888.
Pollini, N., O. Lavan, and O. Amir. 2018. “Optimization-based minimum-cost seismic retrofitting of hysteretic frames with nonlinear fluid viscous dampers.” Earthquake Eng. Struct. Dyn. 47 (15): 2985–3005. https://doi.org/10.1002/eqe.3118.
Rastegaran, M., S. B. Beheshti Aval, and E. Sangalaki. 2022. “Multi-objective reliability-based seismic performance design optimization of SMRFs considering various sources of uncertainty.” Eng. Struct. 261 (May): 114219. https://doi.org/10.1016/j.engstruct.2022.114219.
Razavi, N., and S. Gholizadeh. 2021. “Seismic collapse safety analysis of performance-based optimally designed reinforced concrete frames considering life-cycle cost.” J. Build. Eng. 44 (Aug): 103430. https://doi.org/10.1016/j.jobe.2021.103430.
Razmara Shooli, A., A. R. Vosoughi, and M. R. R. Banan. 2019. “A mixed GA-PSO-based approach for performance-based design optimization of 2D reinforced concrete special moment-resisting frames.” Appl. Soft Comput. J. 85 (Jul): 105843. https://doi.org/10.1016/j.asoc.2019.105843.
Rojas, H. A., C. Foley, and S. Pezeshk. 2011. “Risk-based seismic design for optimal structural and nonstructural system performance.” Earthquake Spectra 27 (3): 857–880. https://doi.org/10.1193/1.3609877.
Rojas, H. A., S. Pezeshk, and C. M. Foley. 2007. “Performance-based optimization considering both structural and nonstructural components.” Earthquake Spectra 23 (3): 685–709. https://doi.org/10.1193/1.2754002.
Saadat, S., C. V. Camp, and S. Pezeshk. 2014. “Seismic performance-based design optimization considering direct economic loss and direct social loss.” Eng. Struct. 76 (Apr): 193–201. https://doi.org/10.1016/j.engstruct.2014.07.008.
Salajegheh, F., E. Salajegheh, and S. Shojaee. 2022. “An enhanced approach for optimizing mathematical and structural problems by combining PSO, GSA and gradient directions.” Soft Comput. 26 (21): 11891–11913. https://doi.org/10.1007/s00500-022-07007-w.
Sanghai, S., and P. Pawade. 2021. “Optimal placement of friction dampers in building considering nonlinearity of soil.” Innov. Infrastruct. Solut. 6 (1): 1–18. https://doi.org/10.1007/s41062-020-00395-8.
Sarcheshmehpour, M., H. E. Estekanchi, and M. A. Ghannad. 2020. “Optimum placement of supplementary viscous dampers for seismic rehabilitation of steel frames considering soil–structure interaction.” Struct. Des. Tall Special Build. 29 (1): 1–17. https://doi.org/10.1002/tal.1682.
Shahnazaryan, D., G. J. O’Reilly, and R. Monteiro. 2022. “On the seismic loss estimation of integrated performance-based designed buildings.” Earthquake Eng. Struct. Dyn. 51 (8): 1794–1818. https://doi.org/10.1002/eqe.3638.
Shin, H., and M. P. Singh. 2014a. “Minimum failure cost-based energy dissipation system designs for buildings in three seismic regions—Part I: Elements of failure cost analysis.” Eng. Struct. 74 (Aug): 266–274. https://doi.org/10.1016/j.engstruct.2014.04.054.
Shin, H., and M. P. Singh. 2014b. “Minimum failure cost-based energy dissipation system designs for buildings in three seismic regions—Part II: Application to viscous dampers.” Eng. Struct. 74 (Apr): 275–282. https://doi.org/10.1016/j.engstruct.2014.05.012.
Shin, H., and M. P. Singh. 2017. “Minimum life-cycle cost-based optimal design of yielding metallic devices for seismic loads.” Eng. Struct. 144 (May): 174–184. https://doi.org/10.1016/j.engstruct.2017.04.054.
Shirkhani, A., B. F. Azar, and M. C. Basim. 2021. “Seismic loss assessment of steel structures equipped with rotational friction dampers subjected to intensifying dynamic excitations.” Eng. Struct. 238 (Jun): 112233. https://doi.org/10.1016/j.engstruct.2021.112233.
Shmerling, A., R. Levy, and A. M. Reinhorn. 2018. “Seismic retrofit of frame structures using passive systems based on optimal control.” Struct. Control Health Monit. 25 (1): e2038. https://doi.org/10.1002/stc.2038.
Sinković, N. L., M. Brozovič, and M. Dolšek. 2016. “Risk-based seismic design for collapse safety.” Earthquake Eng. Struct. Dyn. 45 (9): 1451–1471. https://doi.org/10.1002/eqe.2717.
Spence, S. M. J., and S. Arunachalam. 2022. “Performance-based wind engineering: Background and state of the art.” Front. Built Environ. 8 (Mar): 830207. https://doi.org/10.3389/fbuil.2022.830207.
Spence, S. M. J., and M. Gioffrè. 2012. “Large scale reliability-based design optimization of wind excited tall buildings.” Probab. Eng. Mech. 28 (Apr): 206–215. https://doi.org/10.1016/j.probengmech.2011.08.001.
Spence, S. M. J., and A. Kareem. 2014. “Performance-based design and optimization of uncertain wind-excited dynamic building systems.” Eng. Struct. 78 (Jul): 133–144. https://doi.org/10.1016/j.engstruct.2014.07.026.
Steneker, P., A. Filiatrault, L. Wiebe, and D. Konstantinidis. 2020. “Integrated structural–nonstructural performance-based seismic design and retrofit optimization of buildings.” J. Struct. Eng. 146 (8): 04020141. https://doi.org/10.1061/(ASCE)ST.1943-541X.0002680.
Steneker, P., L. Wiebe, A. Filiatrault, and D. Konstantinidis. 2022. “A framework for the rapid assessment of seismic upgrade viability using performance-based earthquake engineering.” Earthquake Spectra 38 (3): 1761–1787. https://doi.org/10.1177/87552930211065771.
Suksuwan, A., and S. M. J. Spence. 2019. “Performance-based design optimization of uncertain wind excited systems under system-level loss constraints.” Struct. Saf. 80 (Jan): 13–31. https://doi.org/10.1016/j.strusafe.2019.03.004.
Suksuwan, A., and S. M. J. J. Spence. 2018. “Performance-based multi-hazard topology optimization of wind and seismically excited structural systems.” Eng. Struct. 172 (May): 573–588. https://doi.org/10.1016/j.engstruct.2018.06.039.
Taflanidis, A. A., and J. L. Beck. 2009. “Life-cycle cost optimal design of passive dissipative devices.” Struct. Saf. 31 (6): 508–522. https://doi.org/10.1016/j.strusafe.2009.06.010.
Tu, X., Z. He, and G. Huang. 2020. “Performance-based multi-objective collaborative optimization of steel frames with fuse-oriented buckling-restrained braces.” Struct. Multidiscip. Optim. 61 (1): 365–379. https://doi.org/10.1007/s00158-019-02366-9.
Tu, X., Z. He, and G. Huang. 2022. “Seismic multi-objective optimization of vertically irregular steel frames with setbacks upgraded by buckling-restrained braces.” Structures 39 (Aug): 470–481. https://doi.org/10.1016/j.istruc.2022.03.044.
Tubaldi, E., M. Barbato, and A. Dall’Asta. 2016. “Efficient approach for the reliability-based design of linear damping devices for seismic protection of buildings.” ASCE-ASME J. Risk Uncertainty. Eng. Syst. Part A: Civ. Eng. 2 (2): C4015009. https://doi.org/10.1061/AJRUA6.0000858.
Turchetti, F., E. Tubaldi, J. Douglas, M. A. Zanini, and A. Dall’Asta. 2023. “A risk-targeted approach for the seismic design of bridge piers.” Bull. Earthquake Eng. 21 (10): 4923–4950. https://doi.org/10.1007/s10518-023-01717-8.
Vaez, S. R. H., M. A. Fathali, and A. A. Samani. 2024. “An approach for optimum performance-based seismic design of 3D steel moment frames.” Eng. Struct. 301 (Feb): 117248. https://doi.org/10.1016/j.engstruct.2023.117248.
van de Lindt, J. W., and T. N. Dao. 2007. “Evolutionary algorithm for performance-based shear wall placement in buildings subjected to multiple load types.” J. Struct. Eng. 133 (8): 1156–1167. https://doi.org/10.1061/(ASCE)0733-9445(2007)133:8(1156).
Vanderplaats, G. N. 1995. DOT users manual version 4.20, Vanderplaats research & development. Colorado Springs, CO: Vanderplaats Research and Development Inc.
Varaee, H., A. Shishegaran, and M. R. Ghasemi. 2021. “The life-cycle cost analysis based on probabilistic optimization using a novel algorithm.” J. Build. Eng. 43 (Jun): 103032. https://doi.org/10.1016/j.jobe.2021.103032.
Wang, C., B. M. Ayyub, and M. Beer. 2023. “From reliability-based design to resilience-based design.” ASCE-ASME J Risk Uncertainty Eng. Syst. Part B: Mech. Eng. 9 (3): 031105. https://doi.org/10.1115/1.4062997.
Wang, X., Q. Zhang, X. Qin, and Y. Sun. 2020. “An efficient discrete optimization algorithm for performance-based design optimization of steel frames.” Adv. Struct. Eng. 23 (3): 411–423. https://doi.org/10.1177/1369433219872440.
Wang, Y., I. Burgess, F. Wald, and M. Gillie. 2012. Performance-based fire engineering of structures. Boca Raton, FL: CRC Press.
Wen, J., Q. Han, Y. Xie, X. Du, and J. Zhang. 2021. “Performance-based seismic design and optimization of damper devices for cable-stayed bridge.” Eng. Struct. 237 (Jul): 112043. https://doi.org/10.1016/j.engstruct.2021.112043.
Wen, Y. K., and Y. J. Kang. 2001a. “Minimum building life-cycle cost design criteria. I: Methodology.” J. Struct. Eng. 127 (3): 330–337. https://doi.org/10.1061/(ASCE)0733-9445(2001)127:3(330).
Wen, Y. K., and Y. J. Kang. 2001b. “Minimum building life-cycle cost design criteria. II: Applications.” J. Struct. Eng. 127 (3): 338–346. https://doi.org/10.1061/(ASCE)0733-9445(2001)127:3(338).
Xie, Y., and J. Zhang. 2017. “Optimal design of seismic protective devices for highway bridges using performance-based methodology and multiobjective genetic optimization.” J. Bridge Eng. 22 (3): 04016129. https://doi.org/10.1061/(ASCE)BE.1943-5592.0001009.
Xie, Y., and J. Zhang. 2018. “Design and optimization of seismic isolation and damping devices for highway bridges based on probabilistic repair cost ratio.” J. Struct. Eng. 144 (8): 4018125. https://doi.org/10.1061/(ASCE)ST.1943-541X.0002139.
Xu, J., B. F. Spencer, and X. Lu. 2017. “Performance-based optimization of nonlinear structures subject to stochastic dynamic loading.” Eng. Struct. 134 (Apr): 334–345. https://doi.org/10.1016/j.engstruct.2016.12.051.
Xu, L., Y. Gong, and D. E. Grierson. 2006. “Seismic design optimization of steel building frameworks.” J. Struct. Eng. 132 (2): 277–286. https://doi.org/10.1061/(ASCE)0733-9445(2006)132:2(277).
Yaghoobi, S., M. S. Fadali, and G. Pekcan. 2023. “Performance-based active controller design for nonlinear structures using modified black hole optimization.” J. Vib. Control 30 (3–4): 711–726. https://doi.org/10.1177/10775463221150053.
Yazdani, H., M. Khatibinia, S. Gharehbaghi, and K. Hatami. 2017. “Probabilistic performance-based optimum seismic design of RC structures considering soil–structure interaction effects.” ASCE-ASME J. Risk Uncertainty Eng. Syst. Part A: Civ. Eng. 3 (2): 1–12. https://doi.org/10.1061/ajrua6.0000880.
Zacharenaki, A. E., M. Fragiadakis, and M. Papadrakakis. 2013. “Reliability-based optimum seismic design of structures using simplified performance estimation methods.” Eng. Struct. 52 (Apr): 707–717. https://doi.org/10.1016/j.engstruct.2013.03.007.
Zhang, C., and Y. Tian. 2019. “Simplified performance-based optimal seismic design of reinforced concrete frame buildings.” Eng. Struct. 185 (Feb): 15–25. https://doi.org/10.1016/j.engstruct.2019.01.108.
Zhang, J., and R. O. Foschi. 2004. “Performance-based design and seismic reliability analysis using designed experiments and neural networks.” Probab. Eng. Mech. 19 (3): 259–267. https://doi.org/10.1016/j.probengmech.2004.02.009.
Zou, X., Q. Wang, and J. Wu. 2018. “Reliability-based performance design optimization for seismic retrofit of reinforced concrete buildings with fiber-reinforced polymer composites.” Adv. Struct. Eng. 21 (6): 838–851. https://doi.org/10.1177/1369433217733760.
Zou, X. K., and C. M. Chan. 2005. “Optimal seismic performance-based design of reinforced concrete buildings using nonlinear pushover analysis.” Eng. Struct. 27 (8): 1289–1302. https://doi.org/10.1016/j.engstruct.2005.04.001.
Zou, X. K., C. M. Chan, G. Li, and Q. Wang. 2007a. “Multiobjective optimization for performance-based design of reinforced concrete frames.” J. Struct. Eng. 133 (10): 1462–1474. https://doi.org/10.1061/(ASCE)0733-9445(2007)133:10(1462).
Zou, X. K. K., J. G. G. Teng, L. De Lorenzis, and S. H. H. Xia. 2007b. “Optimal performance-based design of FRP jackets for seismic retrofit of reinforced concrete frames.” Composites, Part B 38 (5–6): 584–597. https://doi.org/10.1016/j.compositesb.2006.07.016.
Information & Authors
Information
Published In
Copyright
This work is made available under the terms of the Creative Commons Attribution 4.0 International license, https://creativecommons.org/licenses/by/4.0/.
History
Published online: Jun 10, 2024
Published in print: Aug 1, 2024
Discussion open until: Nov 10, 2024
ASCE Technical Topics:
Authors
Metrics & Citations
Metrics
Citations
Download citation
If you have the appropriate software installed, you can download article citation data to the citation manager of your choice. Simply select your manager software from the list below and click Download.