Indefinability of Effective Stress for Unsaturated Soils
Publication: Journal of Geotechnical and Geoenvironmental Engineering
Volume 150, Issue 8
Abstract
For decades, researchers have searched for an unsaturated soil effective stress that performs analogously to effective stress for saturated soils and provides some simplifications over net stress and suction approaches. A recent debate is whether problems identified with unsaturated soil effective stress can be resolved via elastoplastic analyses. Effective stress equations are reviewed and categorized as being based on volume change, shear strength, yield, or degree of saturation. These equations are evaluated for consistency with saturated soil effective stress expectations using the Barcelona basic model (BBM), simplified into an integrated elastoplastic framework using the modified state surface approach (MSSA). Under isotropic conditions, saturated soil constant volume and yield curves are coincident, and the effective stress principle applies. In contrast, unsaturated soil constant volume and yield curves diverge, which is at root to the indefinability of an effective stress for unsaturated soils. Although effective stress for saturated soils has been defined on the basis of volume change, unsaturated soil effective stress is often defined on shear strength or degree of saturation, with attempted extrapolation to more general volume change and yield responses. Effective stress equations, when used for constitutive modeling of unsaturated soils, cannot recover the form of saturated soil effective stress models, nor provide the often-asserted simplifications or analytical economies. It is demonstrated that volume change and/or yield can occur under any constant unsaturated soil effective stress. It is shown that it is impossible to define an effective stress for unsaturated soils that functions analogously to that of saturated soils. An effective stress approach for unsaturated soils is demonstrated to be, at most, a mathematical transformation, which may provide simplifications for some limited usages.
Introduction
Fredlund (2016) provided a comprehensive discussion on the stress state variable for saturated–unsaturated soils and argued that effective stress is neither an equation, nor a law, nor a constitutive relationship, and it can only be used for saturated soils. However, Khalili et al. (2004, 2022) argued that Bishop’s effective stress, in fact, can explain all unsaturated soil responses, including collapsible soil behavior, if elastoplasticity is recognized. Khalili et al. (2004) presented the following assumptions and assertions in defining effective stress for unsaturated soils: “(1) the state of stress in a material is controlled by only the state of elastic straining, (2) it is sufficient to show that the elastic response is uniquely controlled to demonstrate the effective stress principle, and (3) only wetting and drying stress paths (at constant ) may be considered in validating the effective stress principle. Stress paths considering change in net stress at constant suction need not be considered as in such cases a change in net stress is equal to a change in effective stress and therefore validity of effective stress is guaranteed a priori.” These assertions and assumptions make it clear that their unsaturated soils “effective stress” is different than Terzaghi’s effective stress for saturated soils. After Khalili et al. (2004) suggested elastoplasticity as a justification for use of effective stress for unsaturated soil volume change applications, other researchers have cited this justification, without attention to the restrictive assumptions (e.g., Nuth and Laloui 2008; Lu et al. 2010). Recently, Khalili et al. (2022) reiterated their position that effective stress need only be demonstrated for elastic response, and once demonstrated can be used successfully and directly within an elastoplastic framework. Based on an extensive literature review, Khalili et al. (2022) concluded that effective stress is obtained by establishing a “…mechanical equivalency between a multi-phase system and a [single phase] continuum,… established based on a strain equivalency (e.g., Nur and Byerlee 1971), strength equivalency (Vanapalli et al. 1996; Khalili and Khabbaz 1998) or an energy equivalency (De Boer and Ehlers 1990; Gray and Schrefler 2007; Borja 2006; Einav and Liu 2018).… it must be applicable to all mechanical responses of the soil, e.g. deformation and strength.”
Jommi (2000) pointed out that: ‘‘in fact, no single stress variable has ever been found … for a description of all the aspects of the mechanical behaviour …’’. Gens et al. (2006) concluded that a second variable is required to simulate all unsaturated soil behavior, finding that the most common effective stress approaches used degree (or effective degree) of saturation and suction (or modified suction) (Nuth and Laloui 2008; Gallipoli et al. 2015; Gallipoli and Bruno 2017; Khalili et al. 2022).
Proposed equations of effective stress for unsaturated soils can be found in many state-of-the-art review papers (e.g., Morgenstern 1979; Gens 1995; Delage and Graham 1995; Wheeler and Karube 1995; Sheng 2011; Nuth and Laloui 2008; Kalili et al. 2004, 2022). At least four different definitions have been used for unsaturated soil effective stress: (1) volume change (Bishop 1959; Jennings 1960; Aitchison and Donald 1956; Aitchison 1965; Jennings and Burland 1962; Bishop and Donald 1961; Bishop and Blight 1963; Matyas and Radhakrishna 1968), (2) yield (Wheeler et al. 2003; Lloret Cabot et al. 2013, 2014, 2017), (3) shear/tensile strength (Khalili and Khabbaz 1998; Khalili et al. 2004, 2022; Lu and Likos 2006; Lu et al. 2010), and (4) degree (or effective degree) of saturation (Schrefler 1984; Oberg and Sallfors 1995; Bolzon et al. 1996; Jommi 2000; Gallipoli et al. 2003, 2002; Wheeler et al. 2003; Laloui et al. 2003; Sheng et al. 2004; Tamagnini 2004; Sun et al. 2007a, b, c, 2008; Nuth and Laloui 2008; Romero and Jommi 2008; Lloret Cabot et al. 2013, 2014, 2017).
Herein, the authors review these categories of proposed effective stress equations within an elastoplastic framework for unsaturated soils and explore whether elastoplastic models represent a solution to finding an “effective stress” for unsaturated soils. A two independent (uncombined) stress state variable (net total stress; i.e., net , and suction, ) approach is widely acknowledged as fundamentally sound for explaining all unsaturated soil response (Fredlund and Morgenstern 1977; Alonso et al. 1990; Gens et al. 2006; Gallipoli et al. 2018; Houston 2018; Toll 2020). The modified state surface approach (MSSA) (Zhang and Lytton 2009a, b, 2012; Riad and Zhang 2020, 2022) is the net stress and suction framework used for elastoplastic model simplification, parameter calibration, and explaining behavior under complicated stress paths.
Experimental Data and Model Selection
In this paper, the suction-controlled triaxial tests on a Speswhite kaolin exhibiting collapse response (Fig. 1) of Wheeler and Sivakumar (1995) and Sivakumar (1993) are used. Due to the well-known nature of the Barcelona basic model (BBM), that the BBM was developed for collapse response, and due to commonalities with all unsaturated soil elastoplastic models (Sheng et al. 2004; Gens et al. 2006), the BBM is selected as the net stress and suction elastoplastic vehicle for exploration of attempts to define an “effective stress.” Fig. 1(a) shows averaged results for four isotropic suction-controlled tests by Sivakumar (1993), according to Gallipoli et al. (2010), along with estimated yield points for the four tests (45, 60, 90, and 100 kPa suction, respectively.) Fig. 1(b) shows the deviator stress versus net mean stress at critical states, and Fig. 1(c) presents the values of degree of saturation () on normal compression lines and at critical states (Gallipoli et al. 2003). A well-studied data set on a particular soil is desirable for comparison across different analyses and models. The Wheeler and Sivakumar (1995) data was chosen because (1) the soil exhibits collapsible soil behavior, which has been the primary focus of debates on effective stress for unsaturated soils; (2) the soil has been thoroughly studied by using the BBM; (3) Gallipoli et al. (2010) and Zhang and Xiao (2013) used different approaches and obtained a rare consensus calibration of the BBM (D’Onza et al. 2015; Zhang et al. 2023); and (4) the same data was used to validate effective stress under the various definitions (Khalili et al. 2004; Lu and Likos 2006; Lloret-Cabot et al. 2013; Nuth and Laloui 2008).
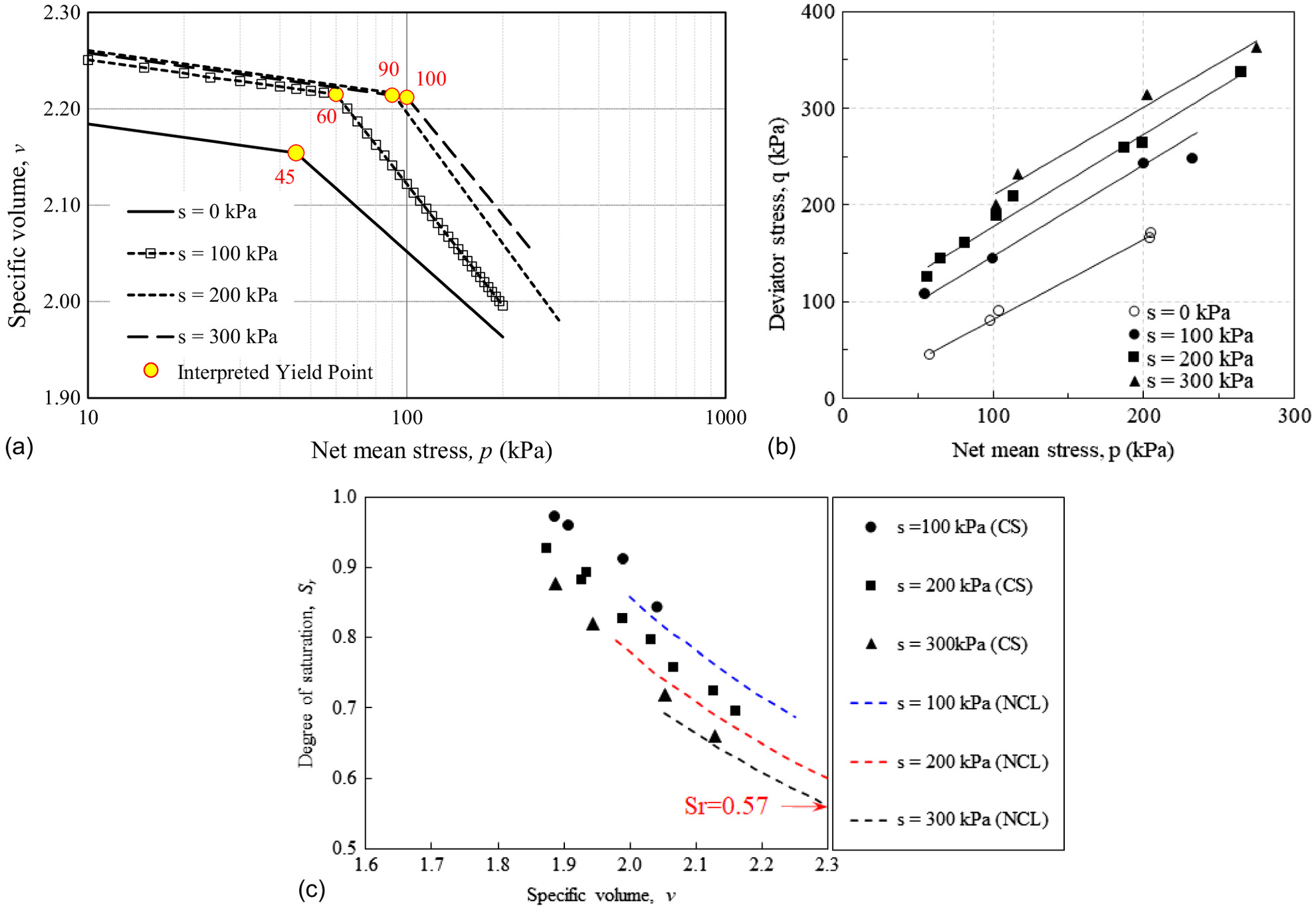
Net Stress and Suction Elastoplastic Framework
MSSA Interpretation of the BBM
The original BBM (Alonso et al. 1990) was developed using traditional incremental formulations. Zhang and Lytton (2009a, b, 2012) developed the MSSA, a unified unsaturated soil volume change framework that provides a smooth bridge between the traditional net stress and suction state surface approach (SSA) and elastoplastic constitutive models for unsaturated soils (Houston and Zhang 2021). The MSSA simplifies analyses for complex incremental elastoplastic unsaturated soil models (Zhang 2016; Zhang et al. 2010, 2023), and has been demonstrated for coupled hydromechanical hysteresis and triaxial loading (Zhang 2010; Zhang and Lytton 2012; Riad and Zhang 2020, 2022). Under isotropic conditions, the MSSA simplifies the BBM into three surfaces as shown in Fig. 2: (1) an elastic surface AFIH; and (2) a plastic surface which is made up of two parts: a plastic collapsible surface FIJG and a plastic expansive surface HIJC, which have the following closed form expressions (see “Notation” for an explanation of the variables):
(1)
(2)
(3)
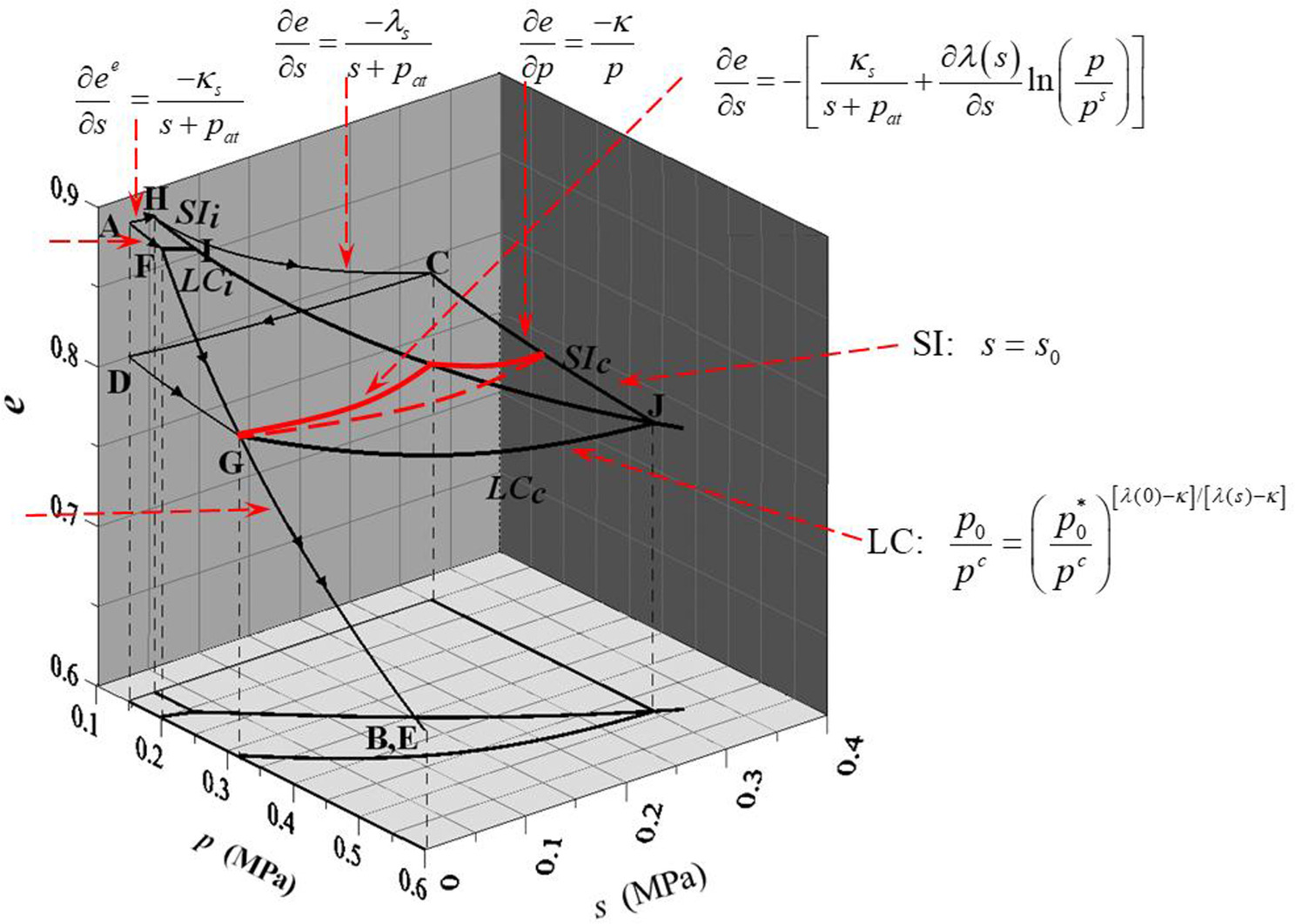
As shown in Fig. 2, the constitutive relations in the BBM are mostly partial derivatives (incremental formulations) of the aforementioned three surfaces and their relationships. Details demonstrating that the MSSA and incremental plasticity methods, including the BBM, are interchangeable can be found in Zhang et al. (2023) and Houston and Zhang (2023). The MSSA clarifies the relationship between the yield curve and the plastic surface (or virgin loading surface); that is, the virgin loading surface is the “future trace” of the yield curve (Zhang and Lytton 2009a, b). The LC yield curve in the BBM is the intersection of the elastic surface and elastoplastic collapsible soil surface. Points on the LC curve must simultaneously satisfy Eqs. (1) and (2), and when the soil is fully saturated, such intersection is the preconsolidation stress, when , giving the yield curve equation in the BBM as follows:
(4)
The suction increase (SI) yield curve can also be obtained in a similar way. However, the SI yield curve has been largely abandoned and is not required or discussed in this paper.
Calibration of the BBM and Yield Curve Determination
Detailed analyses for determining the BBM yield curve and model parameters using the MSSA can be found in Zhang and Xiao (2013) and Zhang et al. (2023). Column Table 1 provides the calibrated model parameters in the BBM by Zhang and Xiao (2013). The results are very close to those obtained from Gallipoli et al. (2010), as shown in Column 3 in Table 1, who used a more complicated approach for BBM calibration. The coefficient of determination () for the analysis is 99.4%, respectively, indicating that the model represents the real soil behavior very well.
Fig. 3(a) shows the Zhang and Xiao (2013) LC yield curve for Fig. 1(a) data. The MSSA does not require establishment of the yield curve during the calibration process because the LC yield curve is simply obtained as the intersection of the unloading–reloading elastic surface [Eq. (1)] and the plastic surface [Eq. (2)]. By selecting specific values of in Eq. (1) so that the yield curves exactly pass through the observed yield points for each compression test, , , , and are found [Fig. 3(a)]. The hardening parameters are calculated as the yield stress at zero suction. It is found that are 45, 24.4, 22.0, and 16.4 kPa for the tests at suctions of 0, 100, 200, and 300 kPa, respectively. The Zhang and Xiao (2013) analyses demonstrate that the normal compression test specimens did not have identical stress histories, and thus the yield points at different suctions do not belong to the same LC yield curve. For comparison, Fig. 3(a) also shows the “traditional” net stress and suction interpretation of the data by Nuth and Laloui (2008), which implicitly and erroneously assumes the same stress history for all four specimens by forcing all four yield points to fall on one yield curve. For comparison here and for subsequent discussion, Fig. 3(b) shows the Nuth and Laloui (2008) yield curves using both the net stress and suction approach and the effective stress approach. As a minor aside point, although air-entry value (AEV) could easily be considered in the BBM (and MSSA), here AEV was assumed to be zero, for consistency with the original BBM and calibrations by Gallipoli et al. (2010) and Zhang and Xiao (2013). However, the conclusions reached in this paper are unchanged by inclusion or noninclusion of a specific AEV.
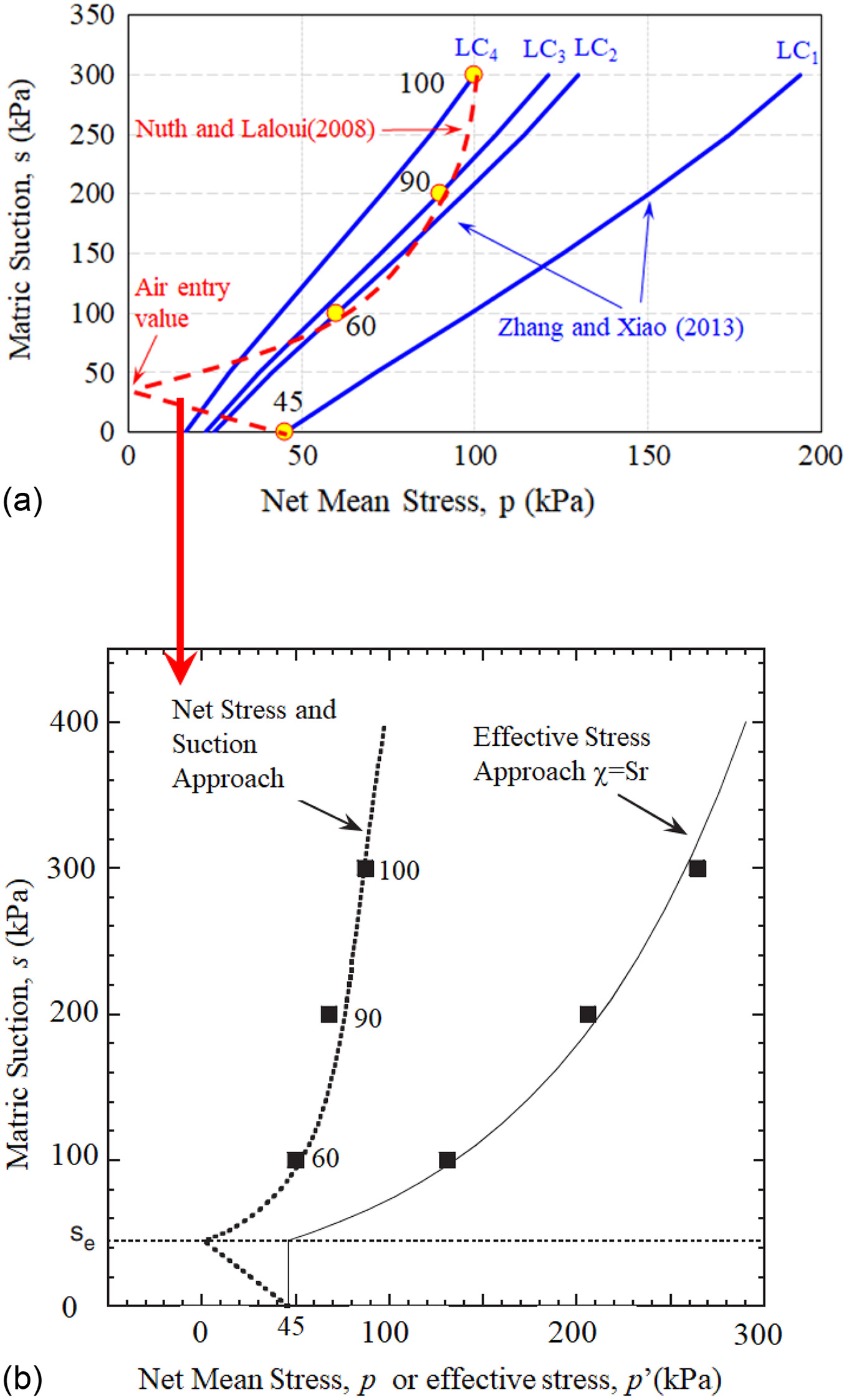
Changes in Specific Volume along the Yield Curve
Fig. 4 shows the changes in specific volume along the four yield curves depicted in Fig. 3(a). The results were obtained by inserting the and combinations along the four yield curves into the BBM elastoplastic surface formulation [Eq. (2)] along with the Table 1 parameters. Specific volume of the soil along any given yield curve decreases as the net stress increases, as expected. Because the yield curve is the border between the elastic and elastoplastic regions, changes in the specific volume along the yield curve can also be calculated by the elastic Eq. (1), using the values and and combinations for any particular yield curve. Along the LC yield curves in Fig. 3(a), suction increases with increase in net stress, and therefore the specific volumes computed using Eq. (1) decrease with increases in both and . Importantly, Fig. 4 demonstrates that the specific volumes along yield curves are not constant. Although this conclusion was obtained for the BBM yield curve shape and the specific soil tested by Sivakumar (1993), in general, specific volume is not constant along yield curves for all existing elasto-plastic models for unsaturated soils, where expansive or collapsible (Zhang and Lytton 2009b; Houston and Zhang 2023).
Exploration of the Calibrated Model
A Simulated Constant Volume Test
Once the model parameters are correctly calibrated, the BBM can be used to predict the soil behavior under different stress paths. Assume that a constant volume test is performed on the Wheeler and Sivakumar (1995) kaolin with an initial condition corresponding to Yield Curve 1, Fig. 5(a). A constant volume path in space, starting from point in the elastic zone [elastic surface ABED in Fig. 5(c)], is shown in Fig. 5(a). Fig. 5(b) shows the specific volume along the stress path, and Fig. 5(c) shows the three-dimensional (3D) MSSA view of the calibrated BBM model and calculated results as shown in Fig. 5(b) for stress path in Fig. 5(a). At point , , and are 0.0252 and 0.3 MPa, respectively, and the corresponding void ratio is 2.205, according to Eq. (1) and the calibrated model (Table 1). Elastic surface ABED in Fig. 5(c) is found, by Eq. (1), to have a of 2.109, and the elastic stress path for is defined as follows:
(5)
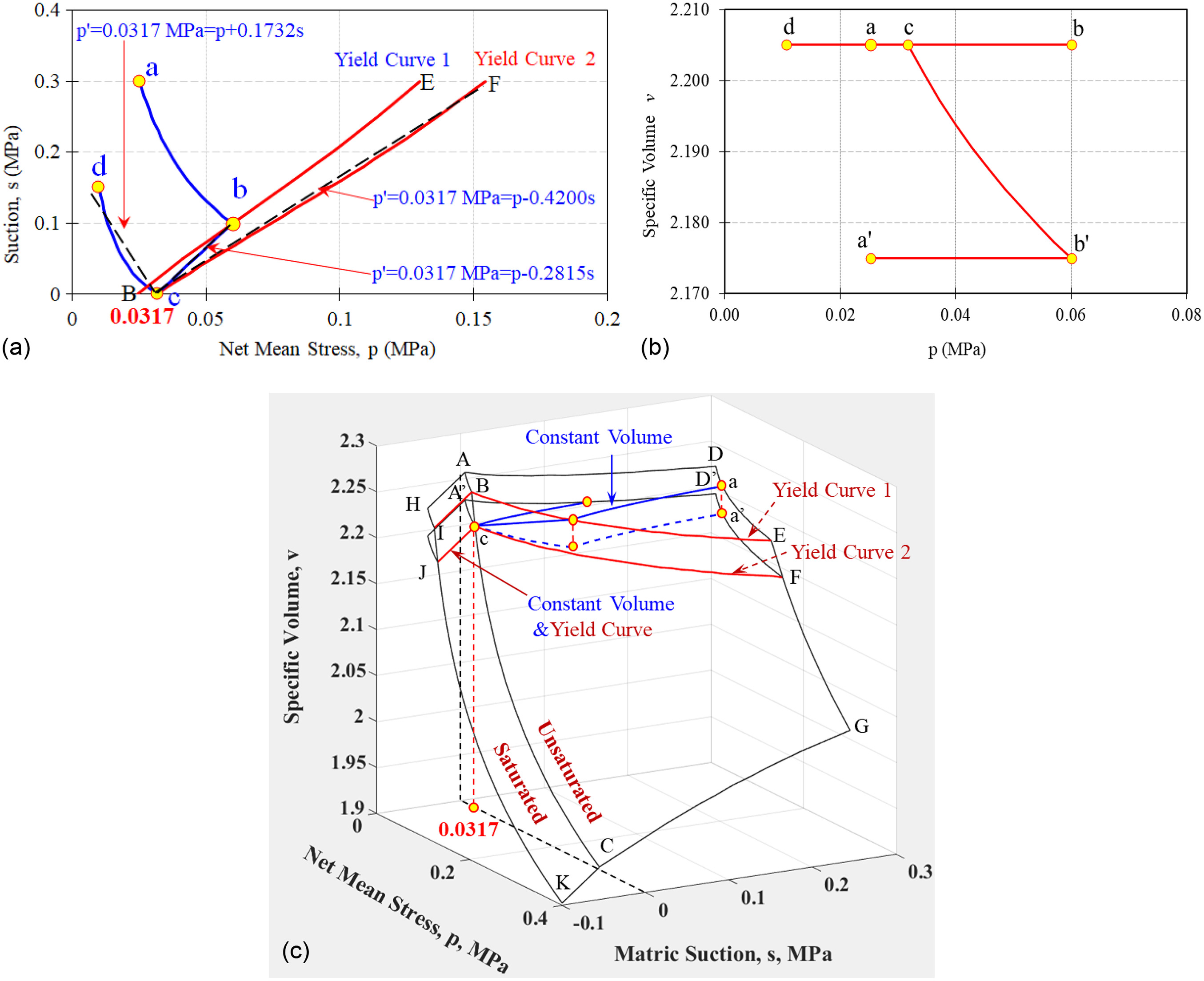
As shown in Fig. 5(b), the specific volume along stress path is a constant. From points (at point , , ), the soil tends to collapse (volume decrease) upon wetting. To maintain a constant volume, must be reduced during the wetting process. The constant volume process from points will be on the elastoplastic surface BCEFG of Fig. 5(c). The expression for stress path can be obtained by inserting the specific volume of 2.205 into the elastoplastic surface Eq. (2)
(6)
At zero suction (point ), the corresponding effective stress (preconsolidation stress) is 0.0317 MPa. From points , the soil experienced yielding and the yield curve moves from position 1 () to 2 (cF) as shown in Figs. 5(a and c), resulting in a new elastic surface , at point along the new yield curve 2. At point , , , and , and using elastic surface Eq. (1), . The new elastic surface in Fig. 5(c) has the following expression:
(7)
The drying process from point is an elastic (unloading) process according to the BBM. The soil volume tends to decrease with suction increase. To keep the specific volume constant, must therefore be reduced as shown by stress path on Fig. 5(a) and on the surface in Fig. 5(c).
An additional test can be run by taking the reverse stress path from in Fig. 5(a). The process is elastic because the whole stress path lies on the left side of the current yield curve . All of the soil responses fall on the same elastic surface as shown in Fig. 5(c) and can be calculated by inputting and for stress paths of () and (a’), into Eq. (7). Specifically, the soil response from is the same as that from because the volume change is recoverable (neglecting hysteresis) in the elastic zone. Along the reverse stress path to there are increases in both suction and net stress in the elastic zone, which will cause decrease in the specific volume as shown in Fig. 5(b). As a result of plastic yielding along constant volume stress path , the specific volume along the stress path remains constant under a combined reduction in and increase in , but at lower volume (2.177) than that along (2.205). The specific soil tested by Sivakumar (1993) demonstrates collapsible soil behavior, and constant volume test data on this or other collapsible soils is not readily available. That yield can occur during constant volume testing has, however, been demonstrated by Zhang and Lytton (2009b) and Houston and Zhang (2021) for unsaturated expansive soil by analyzing the data from Brackley (1975) wherein yield occurs during a constant volume test.
Smooth Transition between Saturated and Unsaturated Conditions
One commonly cited criticism of net stress and suction models, including the BBM, is that a smooth transition between saturated and unsaturated soils is not achieved. This is, however, a misunderstanding. Using the BBM as an example, under isotropic conditions, and at , Eqs. (1) and (2) become
(8)
(9)
Note that for all saturated soil conditions (i.e., below AEV), the effective stress principle holds true, and . Fig. 5(c) shows, for both saturated and unsaturated conditions, the specific volume change of the soil under isotropic conditions. The saturated conditions are represented by surface ABCKJIH. For the real soil of Wheeler and Sivakumar (1995), Fig. 5(c) shows coincidence of constant volume and yield curves for saturated conditions, and divergence of these curves for unsaturated conditions.
Fig. 6 is a schematic of the transition from unsaturated to saturated conditions for real soils in which desaturation occurs at the AEV and the saturated soil effective stress principle holds true for cases when suction is lower than the AEV. Fig. 6 shows the constant volume curve and the yield curve coincident for suction below the AEV, and divergent when the soil becomes unsaturated, whether the soil is expansive or collapsible. The divergence of the constant volume and yield curves for unsaturated soil conditions portends problems in defining an effective stress for unsaturated soils that is analogous to Terzaghi’s effective stress for saturated soils. Indeed, deviation of unsaturated soil “effective” stress equations from the definition of effective stress for saturated soils was specifically acknowledged by Khogo et al. (1993) and Nuth and Laloui (2008).
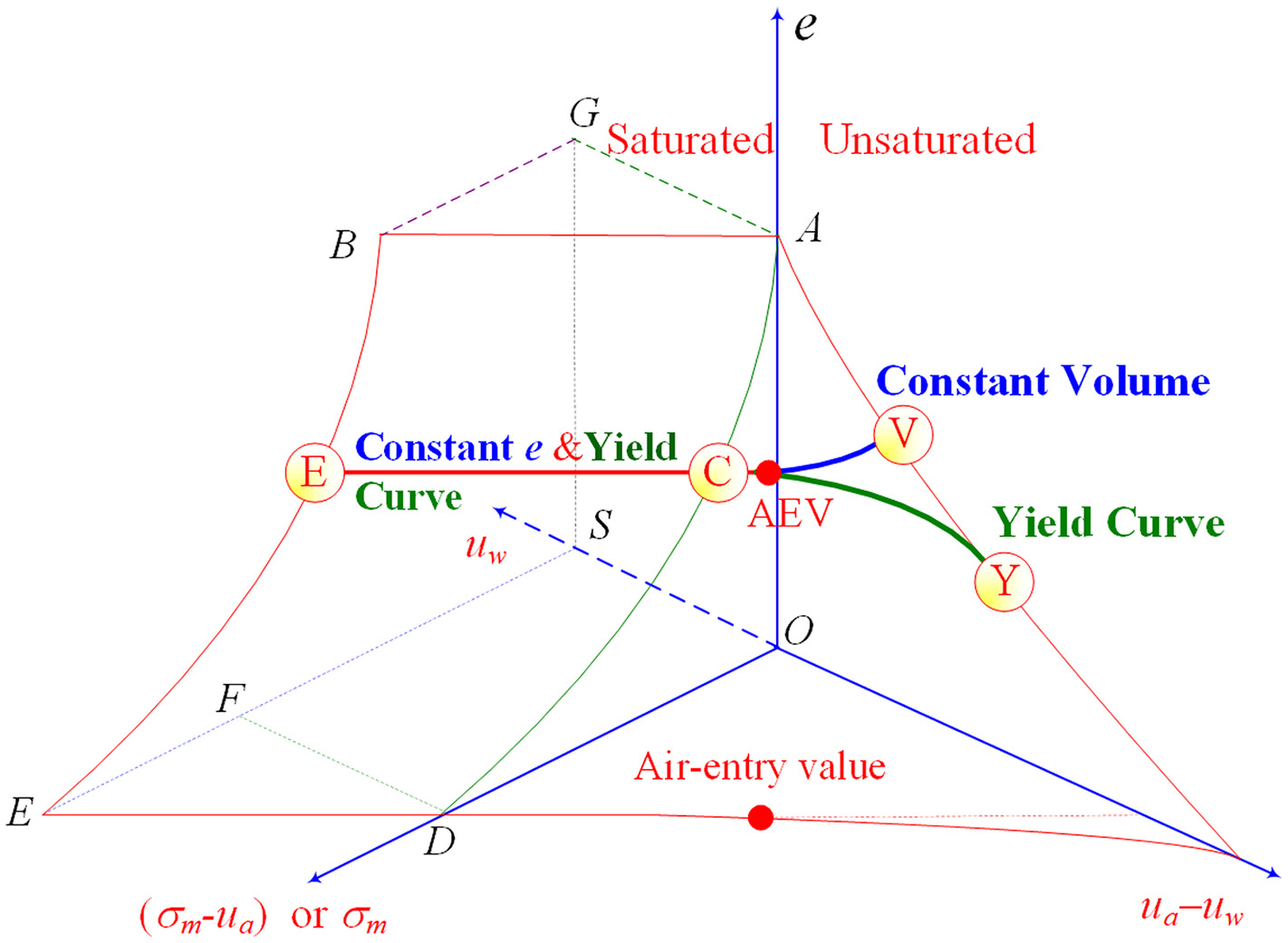
Elastoplastic Review of “Effective Stress” Approaches
Researchers have defined effective stress for unsaturated soils, either explicitly or nonexplicitly, based upon (1) constant volume (Bishop 1959; Aitchison and Donald 1956; Jennings and Burland 1962; Aitchison 1965; Bishop and Donald 1961; Bishop and Blight 1963; Matyas and Radhakrishna 1968), (2) yield (Wheeler et al. 2003; Lloret-Cabot et al. 2013), (3) shear/tensile strength (Khalili and Khabbaz 1998; Khalili et al. 2004, 2022; Lu and Likos 2006), and (4) degree of saturation or (Wheeler et al. 2003; Nuth and Laloui 2008; Lloret-Cabot et al. 2013). For people outside of the unsaturated soil community, effective stress for unsaturated soils is expected to be just like Terzaghi’s effective stress for saturated soils, which is a single stress (equation) that can be used for all soils (expansive, collapsible or both), all soil features (volume change, yielding, shear strength), and all possible stress paths (static and dynamic loading/unloading, cyclic drying and wetting). By considering the constant volume test of Fig. 5, the divergent constant volume and yield curves, and the volume change conditions associated with soil failure (shear strength), the various bases for defining effective stress are evaluated within the context of elastoplastic unsaturated soil response.
Effective Stress for Unsaturated Soils Defined by Constant Volume
Under a constant volume–based definition, if the soil volume associated with the proposed effective stress equations remains constant while and vary, then this combining equation of and is the unsaturated soil effective stress. The magnitude of volume change-based unsaturated soil effective stress is normally obtained by reference to the saturated soil effective stress () (Bishop 1959; Jennings 1960; Aitchison and Donald 1956; Jennings and Burland 1962; Aitchison 1965; Bishop and Donald 1961; Bishop and Blight 1963; Matyas and Radhakrishna 1968).
Taking Fig. 5 as an example, under a constant volume definition, the effective stress along the stress path should be the same because there is no volume change and, further, the effective stress should be equal to 0.0317 MPa as indicated by point at saturation. However, from , the effective stress will change due to the change in soil volume. From , the effective stress will remain constant due to no volume change; however, the effective stress is different from that along the stress path because soil volumes are different, as shown in Figs. 5(b and c).
If the constant volume effective stress definition and a Bishop-type equation is used to develop an elastoplastic model equivalent to the BBM, the solution of in Bishop’s Equation () can be derived by substitution. For example, for stress path , the effective stress is 0.0317 MPa and can be found from the elastic surface Eqs. (1) and (7)
(10)
Similarly, for stress path , the close form solution of in Bishop’s Equation can be derived from Eqs. (2) and (6)
(11)
Fredlund and Morgenstern (1977) pointed out that Bishop’s Equation contains a material parameter , and that the variables used for the description of a stress should be independent of the material properties. Khalili et al. (2004) argued that the theoretical basis for this argument is not clear. Eqs. (10) and (11) demonstrate that are indeed influenced by soil properties such as , , , , and .
Fig. 7 shows the calculated along stress paths and , respectively. As can be seen from Fig. 7, is positive for stress path , varying from 0.144 to 0.397, while is negative for stress path , varying from –0.265 to –0.283. Fig. 5(a) also shows the trendlines for stress paths and obtained by forcing the intercept point to pass through point with an equivalent effective stress 0.0317 MPa, giving the averaged values for the two stress paths of 0.173 and –0.282, respectively.
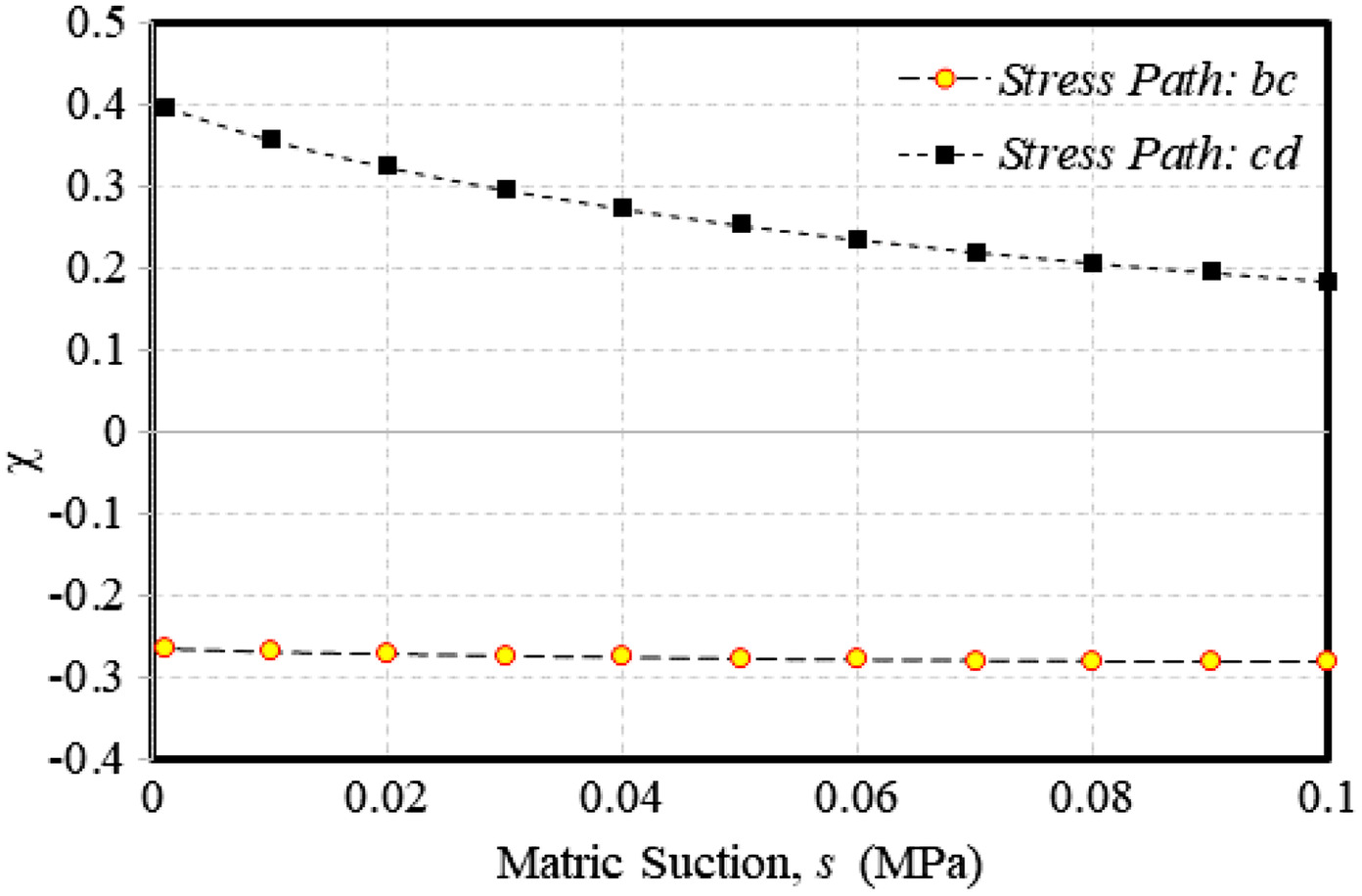
Elastoplastic analyses of the Fig. 5 constant volume test reveal that an unsaturated soil effective stress defined by constant volume has at least four problems:
1.
The effective stress equations will be vastly different in the elastic or elastoplastic zones (e.g., and ), even if the volume is unchanged.
2.
The effective stress is stress path dependent. Points and have the same values of and , yet along constant volume path , effective stress should be constant, while from , effective stress should not be constant because the volume is changing along this path.
3.
The constant volume–based effective stress is stress history dependent. For example, stress paths and are the same in terms of and , and although the computed effective stress along these two paths is the same, the volumes are different because they fall on different elastic surfaces caused by different stress histories.
4.
There is yield along volume change–based constant effective stress paths. For example, for constant volume stress path , effective stress should be constant. However, along stress path , there is yielding, resulting in permanent volume change upon unloading. This deviation further demonstrates the futility of constant volume–based definition of effective stress for unsaturated soils.
Effective Stress for Unsaturated Soils Defined by Yield
A second possible way of defining effective stress for unsaturated soils is no-yield, although such definition deviates from effective stress for saturated soils. A yield-based definition (i.e., soil does not exhibit plastic volume change provided effective stress remains unchanged) was used by some researchers for elastoplastic constitutive modeling of unsaturated soils (e.g., Wheeler et al. 2003; Lloret-Cabot et al. 2013, 2014, 2017; Khalili et al. 2022). Lloret-Cabot et al. (2013) extended the Glasgow coupled model (GCM) (Wheeler et al. 2003) to triaxial stress states. Lloret-Cabot et al. (2017) used the same data of this study (Sivakumar 1993) to validate the GCM. Under a yield-based definition of effective stress, all yield curves become a series of vertical straight lines in space, as shown in Fig. 8(a) (e.g., Wheeler et al. 2003; Lloret Cabot et al. 2013, 2014, 2017; Khalili et al. 2022) and Fig. 8(b). If the yield-based Bishop effective stress definition is used to develop an elastoplastic model equivalent to the BBM, the closed form solution of can be derived from the yield curve Eq. (4)
(12)
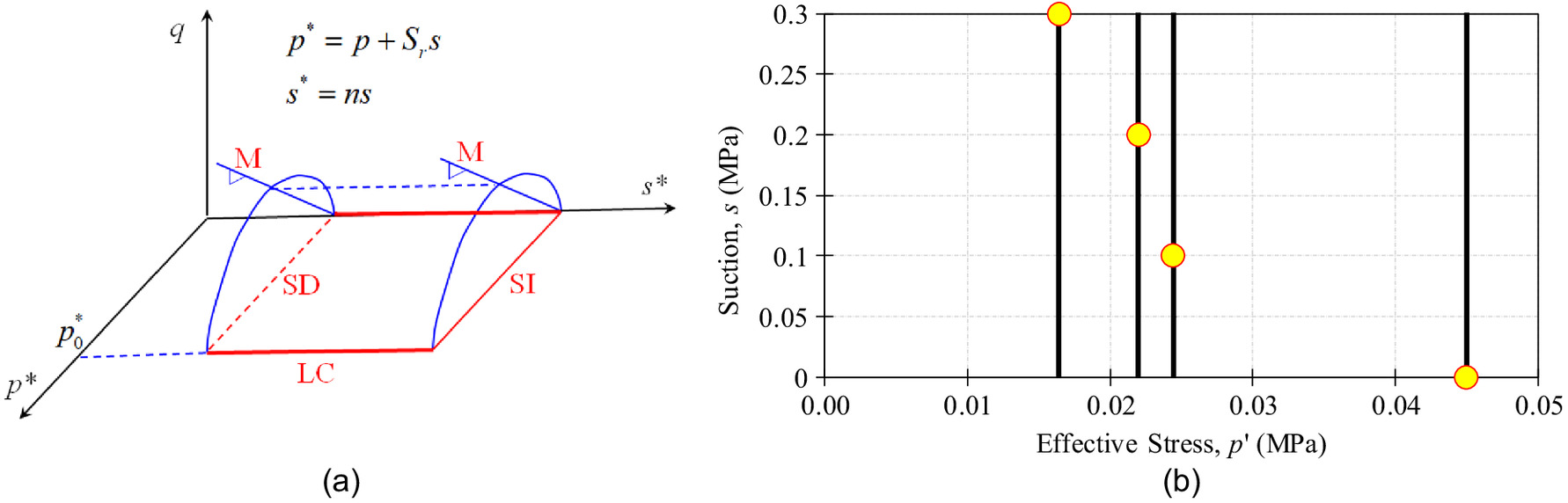
Fig. 8(b) shows the transformed shapes of the four yield curves of Fig. 3(a) in the effective stress [defined by of Eq. (12)] and suction () space, which correspond to preconsolidation stress, , values of 0.045, 0.0244, 0.022, and 0.0164 MPa, respectively. Fig. 9 shows , calculated by Eq. (12) for the four yield curves in Fig. 3(a). As shown in Fig. 9, is between –0.232 and –0.538 along these four yield curves. The constant volume test of Fig. 5(a) also shows negative for yield curve cF (averaged value of –0.42). These demonstrated negative values are inconsistent with the usual Bishop’s-type equations where .
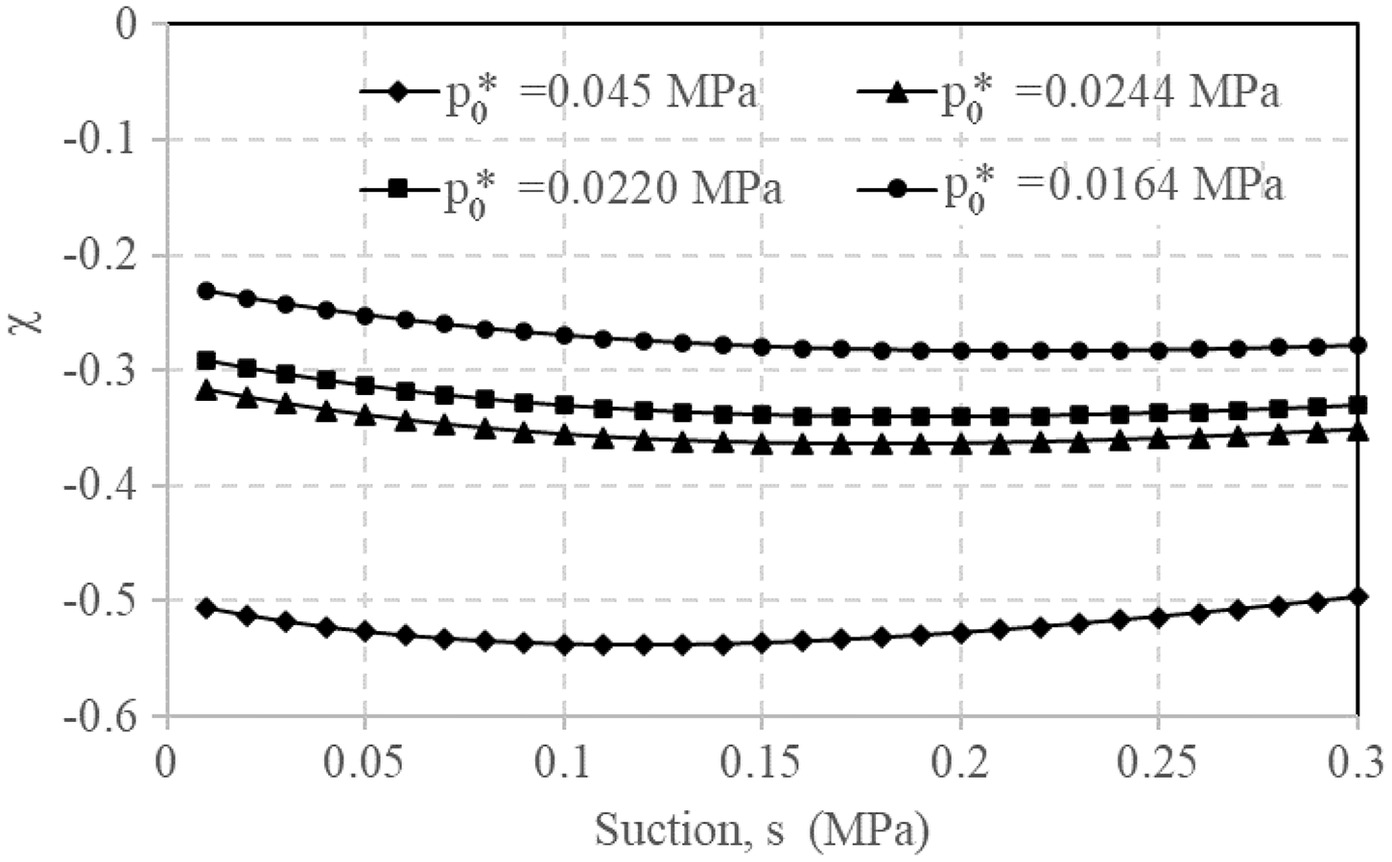
Beyond the negative values, there are at least two problems with a yield-based definition of effective stress for unsaturated soils: (1) volume change will occur under constant effective stress conditions as shown in Fig. 4, conflicting with the well-accepted definition of effective stress for saturated soils; and (2) effective stress cannot be defined in the elastic zone. As clearly demonstrated by the MSSA, the elastoplastic surface represents the “trace” of any future yield curve. For any combination of and resulting in elastoplastic response, a new yield curve is generated on the elastoplastic surface, and the corresponding effective stress is obtained by following the new yield curve to zero suction. Because there is no yielding on the entire elastic surface, a yield-based definition of effective stress offers no basis for obtaining effective stress in the elastic zone, requiring an additional definition of effective stress for elastic soil response.
Effective Stress for Unsaturated Soils Defined by Shear Strength
Contrary to the approach used in the establishment of saturated soil effective stress, many unsaturated soils researchers first developed and validated their effective stress equation for unsaturated soils using shear strength, and subsequently attempted to apply the shear strength–based effective stress to volume change (Khalili and Khabbaz 1998; Khalili et al. 2004, 2022; Lu and Likos 2006; Lu et al. 2010). For shear strength, several empirically fitted effective stress equations fit the available data very well, especially when some degree of nonlinearity is considered by introducing the soil water retention characteristics, as shown in Khalili and Khabbaz (1998), Lu and Likos (2006), and Lu et al. (2010). Typically, the proposed shear strength–based effective stress equations or relationship are extended, without validation, to volume change under the assumption that the unsaturated soil shear strength–based equation is fundamentally based (Khalili and Khabbaz 1998; Lu and Likos 2006). At present, the most used form of the unsaturated soil shear strength equation is that proposed by Fredlund et al. (1978)
(13)
Shear strength is estimated from saturated soil effective stress properties and , together with an empirically established material property coefficients . Eq. (13) is rewritten by some researchers to give the appearance of a Bishop-type effective stress equation with . Eq. (13) is also rewritten in space by Khalili et al. (2004)where is the slope of critical state lines. Khalili and Khabbaz (1998) and Khalili et al. (2004) proposed a nonlinear for Eq. (14), with consideration of AEV. The critical state failure criterion in the BBM is a special case of Eq. (14) with , and as follows:where is a constant describing the increase in cohesion with suction in the BBM (Alonso et al. 1990).
(14)
(15)
Fig. 1(b) shows the deviator stress versus net mean stress at critical states for the compacted Speswhite kaolin tested by Sivakumar (1993). Using Eq. (14), the regression analysis of Fig. 1(b) data indicated the following BBM parameters: , , and . The for the analysis is 99.7%. Fig. 10(a) is a 3D presentation of Fig. 1(b). As can be seen in Fig. 10(a), the process of finding for shear strength tests involves extrapolation of the failure plane to obtain the tensile strength of the soil (when ). Thus, the effective stress proposed by Khalili et al. (2004) and the suction–stress characteristic curve proposed by Lu and Likos (2006) and Lu et al. (2007, 2009) are essentially nonlinear expressions of in the BBM.
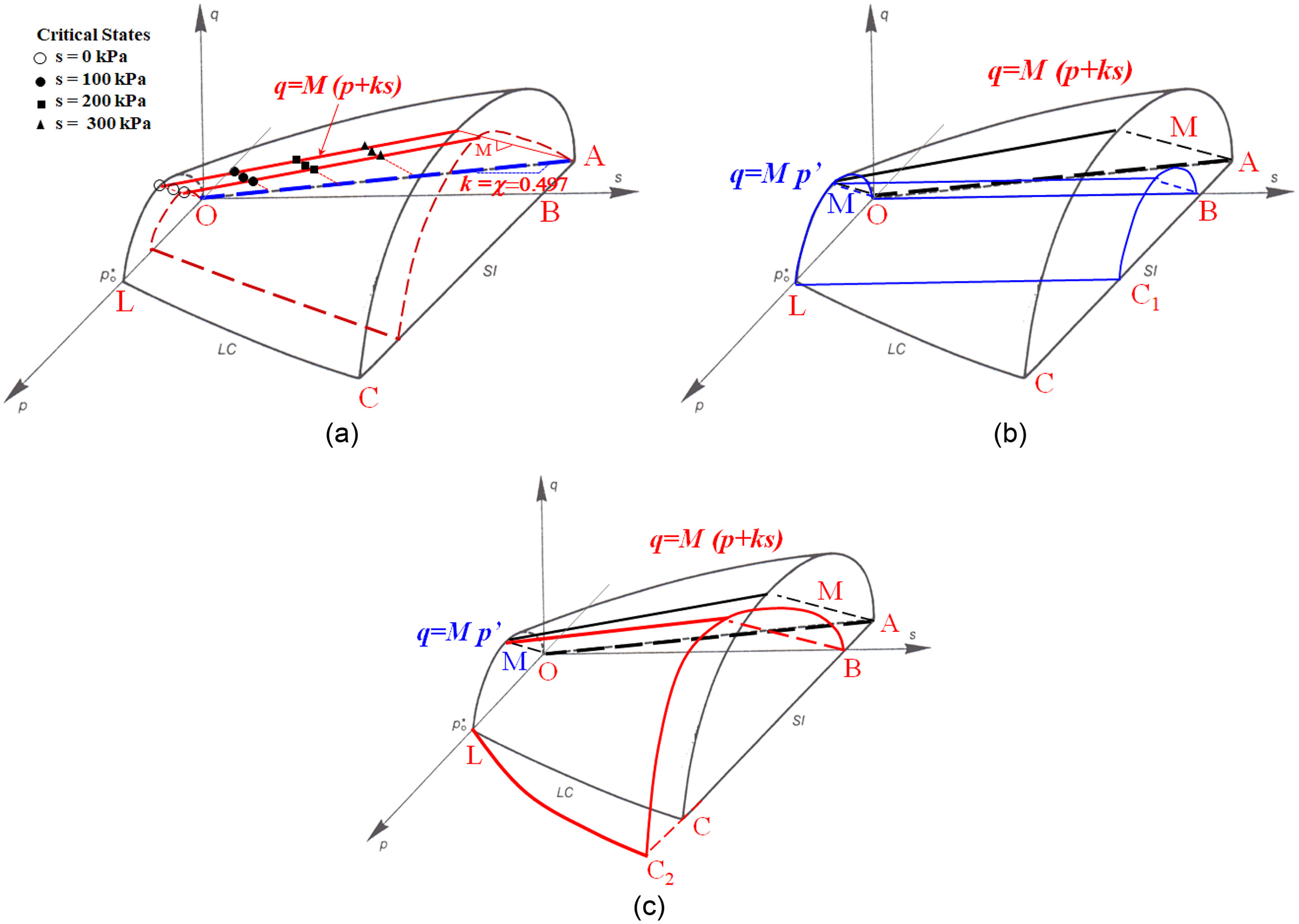
Once the effective stress equation is developed based upon shear strength data for some specific soils, the same equation has been used (extrapolated) to investigate all other features of unsaturated soil response such as volume change and plastic deformation (Khalili and Khabbaz 1998; Khalili et al. 2004, 2022; Lu and Likos 2006). For example, when is applied to Eq. (15), it becomes (Khalili et al. 2022)
(16)
Eq. (16) is the same as the failure criterion of the modified Cam-clay model for saturated soils (Roscoe and Burland 1968). Khalili et al. (2022) then erroneously asserted that when is applied under saturated soil condition, the yield surface of unsaturated soils [Eq. (2) BBM surface, OACL, Fig. 10(a)] will be identical to that for saturated soils, which is equivalent to OACL transforming into the tube shape of , Fig. 10(b). Although yield curves are acknowledged to depend on due to change in preconsolidation pressure, the claim is that the yield surface is a function of only, but independent of suction (Khalili et al. 2004, 2022; Lu et al. 2010), which is similar to the implicit assumption associated with the Fig. 8(a) yield surface of the GCM (Wheeler et al. 2003; Lloret-Cabot et al. 2013, 2014, 2017; Khalili et al. 2022). Of course, if adoption of an unsaturated soil effective stress equation actually did result in an unsaturated soil yield surface shape identical to that of saturated soil, significant modeling simplification would be realized because all of the previous experiences and developments for saturated soils can be directly used for unsaturated soils. But this is not the case, as subsequently demonstrated.
The effective stress equation for the Sivakumar (1993) soil] is developed by extrapolating the failure surface in Fig. 10(a) to (tensile strength) states and can only be applied to that specific condition. When this effective stress equation with positive is applied to the BBM LC yield curve, it will transform the LC in Fig. 10(a) into in Fig. 10(c). This has been correctly demonstrated by Nuth and Laloui’s (2008) “effective stress approach” analysis for the same soil as shown in Fig. 3(b) ( is taken as ; please see the following discussions for effective stress definition). To transform LC into a vertical yield line [ in Fig. 10(b)], negative values are needed as shown in Fig. 9. For example, an average is required to transform the yield curve in Fig. 5(a) into a vertical-line yield curve with effective stress equal to 0.0317 MPa. However, when a negative value is used at OA (tensile strength), it will push OA further away from the asserted OB, and the tube shape of , claimed by Khalili et al. (2022) and Lloret-Cabot et al. (2017) [as shown in Fig. 8(a)], will not be obtained.
As a result, when the “expected” tube-shape saturated soil–type yield surface in Fig. 10(b) is used (e.g., Wheeler et al. 2003; Khalili et al. 2022), it will lead to erroneous soil response. When the correct suction-dependent yield surface in Fig. 10(c) is used [e.g., Nuth and Laloui 2008, Fig. 3(b), effective stress interpretation], the analysis will be more complicated than the net stress and suction approach because transformations from the space to the space (or vice versa) are added requirements. Thus, the use of an effective stress approach is purely a mathematical transformation without any physical meaning, and none of the benefits of effective stress that are commonly asserted can be achieved (Khalili et al. 2022; Gens et al. 2006).
It is also relevant to explore the parameter under general volume change conditions for a shear strength–based effective stress definition. For the Sivakumar (1993) shear strength data, it was found that , which is completely out of the ranges of the values calculated for volume change (Figs. 7 and 9). This is expected because the critical states in Fig. 1(c) are significantly different stress states from that of the Fig. 1(a) isotropic compression tests. Shear strength and volume change are completely different aspects of soil response, with shear strength (failure) state representing only a tiny subset of the more general volume change response.
There are at least four problems associated with effective stress in unsaturated soils defined by shear strength:
1.
Shear strength represents only a small fraction of all soil volume change responses, as illustrated in Fig. 10(a). It is illogical and destined for failure to extrapolate effective stress defined by shear strength at a very specific failure stress state to predict soil volume change behavior at arbitrary stress states.
2.
The determination of shear strength is subjective, and analyses of shear strength data lack rigor, being primarily empirical.
3.
Calibration of the empirical effective stress equation requires soil-specific, laboratory-determined properties, such as the AEV (Khalili et al. 2004) or the soil water retention curve (SWRC) (Lu and Likos 2006). Consequently, equations or relationships developed for one soil cannot be applied to another without laboratory-based calibration.
4.
Under constant shear strength–based effective stress definition, both volume change and yielding occur. This is evident because the back-calculated for shear strength falls outside the range calculated for constant volume–based effective stress (Fig. 7) and yield effective stress (Fig. 9) definitions.
Degree of Saturation–Based Effective Stress for Unsaturated Soils
For a degree of saturation–based effective stress, most researchers used Bishop’s equation with in constitutive modeling of unsaturated soils (Schrefler 1984; Oberg and Sallfors 1995; Bolzon et al. 1996; Jommi 2000; Gallipoli et al. 2002, 2003; Wheeler et al. 2003; Laloui et al. 2003; Sheng et al. 2004; Tamagnini 2004; Sun et al. 2007a, b, c, 2008; Nuth and Laloui 2008; Romero and Jommi 2008). The study by Nuth and Laloui (2008) on the Wheeler and Sivakumar (1995) data serves as an illustrative example of a degree of saturation–based effective stress definition. For the Wheeler and Sivakumar (1995) data, Fig. 1(c) shows values by Gallipoli et al. (2003), and Fig. 11 summarizes the range of values for different Bishop-type effective stress definitions based upon the calibrated parameters of Zhang and Xiao (2013) in Table 1 for the and ranges of 0–300 kPa. The methods used to compute for each definition of effective stress have been previously presented: for constant volume definition, Eqs. (10) and (11) are used; for yield curve definition, Eq. (12) is used; for shear strength definition, Eq. (16) is used; for degree of saturation definition, Fig. 1(c) is used with . As can be seen from Figs. 1(c) and 11, the varies from 0.57 to 1.0 for normal compression lines and 0.66 to 1.0 for critical state lines, and are beyond the ranges of back-calculated for definitions based upon constant volume (elastic varies from 0.14 to 0.40, elastoplastic from –0.26 to –0.28), yield ( from –0.22 to –0.54), and shear strength (). Further, there was no overlap between the values obtained from the various definitions of effective stress, indicating that there is no single definition of that can be used to represent all soil behavior (volume change, yielding, shear strength). As a result, one cannot extend the obtained from one definition of unsaturated soil effective stress to another.
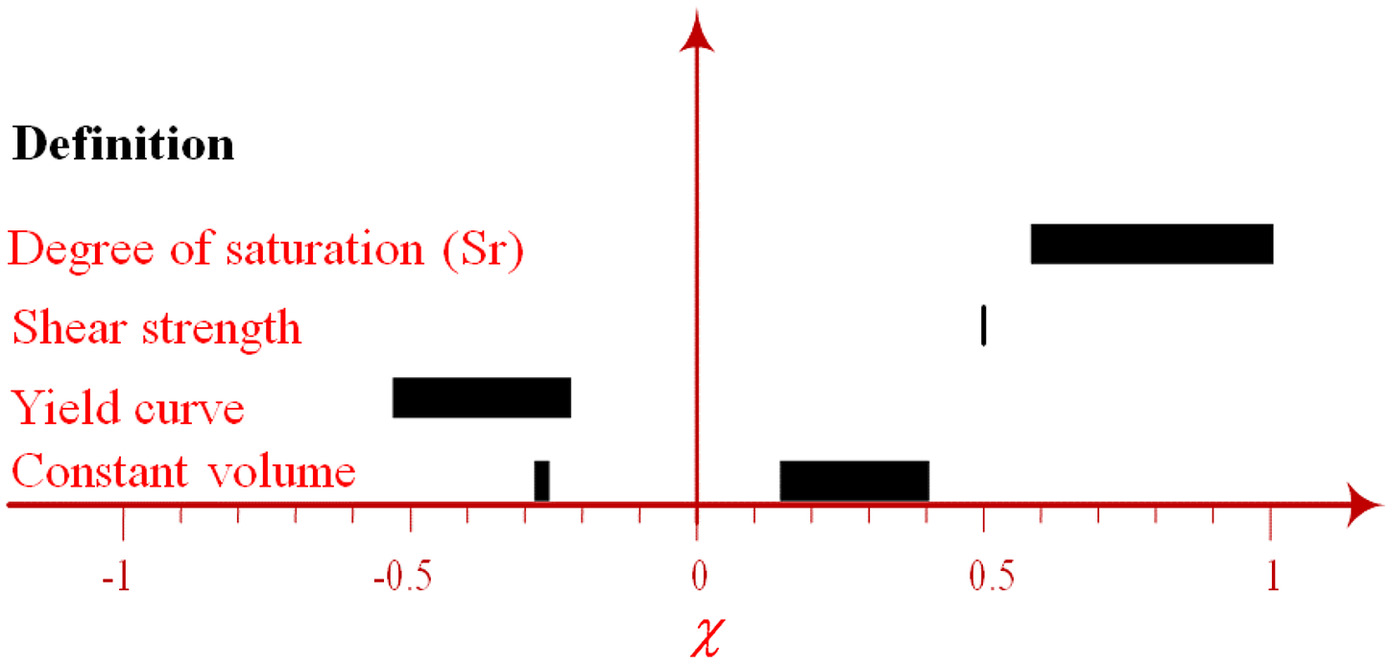
It is crucial to emphasize that the aforementioned issues are not specific to the effective stress definition proposed by Nuth and Laloui (2008), which is taken here as an illustrative example of a degree of saturation–based definition. Instead, challenges and inconsistencies with the established definition of effective stress for saturated soils are inherent in all Bishop-type effective stress–based analyses, including SWRC-based interpretations, whether is linear or nonlinear concerning suction and net stress. Presently, most researchers utilize a Bishop’s equation or other straightforward effective stress equations that constrain their or equivalent within the range of 0 and 1, aligning with the degree of saturation or effective degree of saturation. However, as depicted in Figs. 7 and 9, values surpass the conventional range of 0 to 1. To effectively model collapsible soil behavior using an unsaturated soil effective stress with a defined physical meaning (i.e., either no volume change or no yielding), it is imperative to incorporate negative values for , which makes the degree of saturation–based definition inappropriate.
One often-cited advantage of the Bishop-type effective stress with is that the stress state variables can smoothly transition to saturated effective stress. As demonstrated previously in Fig. 6, the smooth transition can be easily achieved when a net stress and suction approach is used. Adopting separate stress variables of total stress and pore water pressure under saturated conditions is desirable for the accomplishment of smooth transitions where such transitions are important to an application.
Another frequently asserted benefit of Bishop-type effective stress with is its advantages in addressing hysteresis and hydraulic effects in hydromechanical models (Nuth and Laloui 2008; Wheeler et al. 2003; Lloret-Cabot et al. 2013, 2014, 2017). However, this argument directly stems from the common assumption made by most researchers that the degree of saturation is solely a function of suction (Wheeler et al. 2003; Sheng et al. 2008; Vaunat et al. 2000). In contrast, abundant experimental data suggests that the degree of saturation is also influenced by mechanical stress (e.g., Zakaria 1995). When the influence of is taken into account, the use of the Bishop-type effective stress with or becomes significantly disadvantageous because a complex elastoplastic constitutive formulation is required in the determination of . Ultimately, one has to define the degree of saturation as follows:
(17)
This results in an implicit and self-iterative model for the degree of saturation, rendering the modeling of coupled hydro-mechanical behavior of unsaturated soils unnecessarily more complex. In contrast, Riad and Zhang (2022) demonstrated that both mechanical and hydraulic hysteresis can be readily simulated with the net stress and suction approach.
Relationship between the Shape of the Yield Curve and Stress Variables
Another important point that needs attention is the interdependence between the shape of the yield curve and the definition of effective stress when utilizing the effective stress approach. Once the effective stress is defined, the shape of the yield curve, an inherent soil property, becomes determinate for a specific unsaturated soil. For instance, implementing the net stress and suction approach gives yield curves with the shapes depicted in Fig. 3(a) for the Wheeler and Sivakumar (1995) soil. Alternatively, utilizing Eq. (12) (yield-based definition) results in vertical yield curves with shapes as illustrated in Fig. 8(b). Similarly, adopting Bishop’s equation with to define effective stress, as presented in Nuth and Laloui (2008), aligns the shapes (although not the positions, due to differing stress histories) of yield curves more closely with the configuration, as demonstrated in Figs. 10(c) and 3(b), where is positive ().
Consequently, it is erroneous to simultaneously define any effective stress equation and the shape of the yield curve. While this conclusion may seem evident, it is easily overlooked. For instance, in the GCM (Wheeler et al. 2003; Lloret Cabot et al. 2013, 2014, 2017, 2023), which garnered extensive attention, effective stress is defined with (i.e., ), while the yield curves are specified as vertical (LC) and horizontal (SI and SD) straight lines (effectively a yield-based definition of effective stress). Simultaneous definition of effective stress and yield curve are inconsistent, yielding unrealistic and artificial soil properties that do not accurately reflect the actual soil response. Similar problems exist for Khalili et al. (2004, 2022) and Lu and Likos (2006) where an unsaturated soil effectives stress was defined on shear strength, while requiring/expecting the yield surface of the unsaturated soils to be the same as saturated soils.
Even if collapsible soil behavior can be simulated using an effective stress approach with the correct yield surface, similar to Nuth and Laloui (2008) [Fig. 3(b)], it only demonstrates that “a second variable is generally required to represent the stabilizing influence of suction on intergranular forces and the volumetric effects of its removal or weakening, by wetting (Gens et al. 2006).” It does not prove that the effective stress for unsaturated soils carries the same physical meanings as the effective stress for saturated soils as originally proposed by Terzaghi (1936).
Discussion and Conclusions
The challenge in using an effective stress approach for the modeling of unsaturated soils lies in the formidable task of finding a simple equation that can (1) meet the criteria of no volume change and no yielding under constant effective stress; and (2) remain consistent with Terzaghi’s effective stress principles. Effective stress for saturated soils was first proposed and validated for volume change, and subsequently found to be valid for shear strength as well. This is reasonable because the requirements for an effective stress to hold for volume change are more rigorous than for shear strength, because a soil can initially behave elastically, enter the elastoplastic zone with increase stress, and finally fail at a certain critical deviatoric stress state. The critical states are a tiny subset of the more general volume change response. Speculatively, if a volume change–based effective stress for unsaturated soils could be identified, such effective stress might be expected to be applicable for shear strength as well. Saturated soil effective stress principles apply because the constant volume and yield curves coincide for isotropic conditions. Unfortunately, for unsaturated soils, the constant volume and yield curves are divergent, rendering the search for a Terzaghi-equivalent definition of effective stress impossible.
In spite of saturated soil effective stress being volume change based, a review of effective stress equations for unsaturated soils reveals definitions that fall within four different bases: (1) volume change, (2) yield, (3) shear strength, and (4) degree of saturation. Problems within each category of effective stress definition have been demonstrated within an elastoplastic framework. In summary, the following problems are identified:
•
Volume change: Using a Bishop-type effective stress equation, values are demonstrated to be both stress path and stress history dependent, varying from positive to negative along constant volume paths. Specimen yield occurs along constant effective stress paths.
•
Yield: Volume change will occur under constant no-yield effective stress, and a yield definition cannot be used within the elastic zone.
•
Shear-strength: Under constant shear strength–based effective stress, both volume change and yielding occur. Equations developed for one soil cannot be applied to another without laboratory-based calibration.
•
Degree of saturation: Both volume change and shear strength change occur under constant degree of saturation effective stress. Degree of saturation is outside of the range of values determined for all other effective stress definitions. For hydraulic coupling, or becomes significantly disadvantageous because a complex elastoplastic formulation is required to determine due to mechanical stress effects.
While the BBM was used as a conduit for discussions, similar conclusions apply to all existing unsaturated soil elastoplastic models because of the divergence of constant volume and yield curves and the impossibility of extrapolating shear strength–based effective stress to the volume change and yield response. Effective stress equations, when used for constitutive modeling of unsaturated soils, cannot recover the form of saturated soil effective stress models, nor provide the often-asserted simplifications and economies.
It is exceedingly difficult to prove an unsaturated soil effective stress equation, requiring validity across all soils and all possible stress paths, while maintaining the same physical meaning as Terzaghi’s effective stress. Conversely, disproving an equation as the effective stress for unsaturated soils is relatively straightforward, requiring just one counterexample. This study used the widely cited experimental data by Wheeler and Sivakumar (1995) to demonstrate that it is impossible to define an effective stress for unsaturated soils that can function the same as saturated soil effective stress. This is not surprising given that saturation is a special condition of unsaturated soils, and conclusions drawn for a specific case often do not apply in general.
At present, all researchers used suction-controlled (or suction measured) triaxial tests to characterize unsaturated soils in which the net mean stress and suction are independently controlled/measured. A Bishop’s stress variable cannot be determined without first performing net stress and suction controlled/measured laboratory testing. The authors agree with the well-accepted concept that two stress variables are needed to fully represent unsaturated soil behavior (Gens et al. 2006), while arguing that the net stress and suction approach is a more convenient way for modeling, even where transition from saturated to unsaturated conditions is required. The Bishop-type equation can be used in conjunction with a second variable for the modeling of unsaturated soils. However, the fundamental theory of unsaturated soil mechanics should be presented based on the experimentally validated controlling stress state variables of net stress and suction.
Bishop’s-type equations should not be called “effective stress” because this terminology is misleading due to the effective stress for unsaturated soil being indefinable, as demonstrated herein. A similar opinion regarding this misleading terminology was expressed by Kohgo et al. (1993) and Nuth and Laloui (2008). Terminology is not a triviality because the geotechnical community at-large has come to expect a certain meaning of the term “effective stress”—a meaning that does not apply to any unsaturated soil effective stress equations. Mainstream adoption of unsaturated soil mechanics is facilitated by clear communication that there is no effective stress that converts this three-phase media into a mechanically equivalent single-phase continuum.
Notation
The following symbols are used in this paper:
- , , , and
- constants;
- in the BBM = a constant;
- void ratio;
- parameter that relates cohesion and suction;
- slope of theoretical critical state line, ;
- specific volume for ;
- specific volume for ;
- = net mean stress;
- = mean effective stress for saturated soils, ;
- atmospheric pressure;
- apparent preconsolidation pressure at a certain suction;
- preconsolidation pressure in saturated conditions;
- = deviatoric stress;
- parameter controlling the slope of the virgin compression line;
- degree of saturation;
- effective degree of saturation;
- = soil suction, where is the air pressure and is the water pressure;
- specific volume;
- parameter that controls the slope of the virgin compression line for ;
- volumetric strain;
- slope of the unloading–reloading line associated with the mean net stress;
- slope of the unloading-reloading line associated with soil suction;
- slope of the virgin compression line associated with soil suction;
- slope of the virgin compression line associated with the mean net stress for ; ;
- slope of the virgin compression line associated with the mean net stress for ;
- effective stress parameter or Bishop’s parameter quantifying the contribution of suction to the effective stress of the solid skeleton; and
- and
- empirically established material property coefficients.
Data Availability Statement
The data supporting the findings of this study are available from the corresponding author upon request. Researchers interested in accessing the data for the purpose of replication, verification, or further analysis may contact the corresponding author to obtain the necessary information.
Acknowledgments
The authors gratefully acknowledge the financial support provided by the National Science Foundation (NSF) under Grant 2229380. The invaluable support and resources provided by the NSF are highly appreciated.
References
Aitchison, G. D. 1965. “Soil properties, shear strength, and consolidation.” In Proc., 6th Int. Conf. SMFE, 319–321. Toronto: Univ. of Toronto Press.
Aitchison, G. D., and I. B. Donald. 1956. “Some preliminary studies of unsaturated soils.” In Proc., 2nd ANZ Conf. SMFE, Technical Publication, 192–199. Wellington, New Zealand: New Zealand Institution of Engineers.
Alonso, E. E., A. Gens, and A. Josa. 1990. “A constitutive model for partially saturated soils.” Géotechnique 40 (3): 405–430. https://doi.org/10.1680/geot.1990.40.3.405.
Bishop, A. W. 1959. “The principle of effective stress.” Teknisk Ukeblad 106 (39): 859–863.
Bishop, A. W., and G. E. Blight. 1963. “Some aspects of effective stress in saturated and unsaturated soils.” Géotechnique 13 (Mar): 177–197. https://doi.org/10.1680/geot.1963.13.3.177.
Bishop, A. W., and I. B. Donald. 1961. “The experimental study of partly saturated soil in triaxial apparatus.” In Proc., 5th Int. Conf. SMFE, Dunod, Paris, 13–21. London: International Society for Soil Mechanics and Geotechnical Engineering.
Bolzon, G., B. A. Schrefler, and O. C. Zienkiewicz. 1996. “Elastoplastic soil constitutive laws generalized to partially saturated states.” Géotechnique 46 (2): 279–289. https://doi.org/10.1680/geot.1996.46.2.279.
Borja, R. I. 2006. “On the mechanical energy and effective stress in saturated and unsaturated porous continua.” Int. J. Solids Struct. 43 (Mar): 1764–1786. https://doi.org/10.1016/j.ijsolstr.2005.04.045.
Brackley, I. J. A. 1975. “Swell under load.” In Vol. 1 of Proc., 6th Regional Conf. for Africa Soil Mechanics and Foundation Engineering, Durban, South Africa. National Building Research Institute, CSIR, Pretoria, South Africa, 65–70. Cape Town, South Africa: A. A. Balkema.
De Boer, R., and W. Ehlers. 1990. “The development of the concept of effective stresses.” Acta Mech. 83 (1–2): 77–92. https://doi.org/10.1007/BF01174734.
Delage, P., and I. Graham. 1995. “State of the art report-understanding the behavior of unsaturated soils requires reliable conceptual models.” In Vol. 3 of Proc., 1st Int. Conf. on Unsaturated Soils, Paris, 1323–1356. Rotterdam, Netherlands: A. A. Balkema.
D’Onza, F., S. J. Wheeler, D. Gallipoli, M. B. Bucio, M. Hofmann, M. Lloret-Cabot, A. L. Morancho, C. Mancuso, J.-M. Pereira, and E. R. Morales. 2015. “Benchmarking selection of parameter values for the Barcelona basic model.” Eng. Geol. 196 (Sep): 99–118. https://doi.org/10.1016/j.enggeo.2015.06.022.
Einav, I., and M. Liu. 2018. “Hydrodynamic derivation of the work input to fully and partially saturated soils.” J. Mech. Phys. Solids 110 (Jan): 205–217. https://doi.org/10.1016/j.jmps.2017.10.004.
Fredlund, D. G. 2016. “State variables in saturated-unsaturated soil mechanics.” Soil Rocks 39 (1): 3–17.
Fredlund, D. G., and N. R. Morgenstern. 1977. “Stress state variables for unsaturated soils.” J. Geotech. Eng. Div. 103 (5): 447–466. https://doi.org/10.1061/ajgeb6.0000423.
Fredlund, D. G., N. R. Morgenstern, and R. A. Widger. 1978. “Shear strength of unsaturated soils.” Can. Geotech. J. 15 (3): 313–321. https://doi.org/10.1139/t78-029.
Gallipoli, D., and A. W. Bruno. 2017. “A bounding surface compression model with a unified virgin line for saturated and unsaturated soils.” Géotechnique 67 (8): 703–712. https://doi.org/10.1680/jgeot.16.P.145.
Gallipoli, D., A. W. Bruno, F. D’onza, and C. Mancuso. 2015. “A bounding surface hysteretic water retention model for deformable soils.” Géotechnique 65 (10): 793–804. https://doi.org/10.1680/jgeot.14.P.118.
Gallipoli, D., F. D’Onza, and S. J. Wheeler. 2010. “A sequential method for selecting parameter values in the Barcelona basic model.” Can. Geotech. J. 47 (11): 1175–1186. https://doi.org/10.1139/T10-017.
Gallipoli, D., A. Gens, J. Vaunat, and E. Romero. 2002. “Role of degree of saturation on the normally consolidated behaviour of soils.” In Proc., 3rd Int. Symp. on Unsaturated Soil, 115–120. Rotterdam, Netherlands: A. A. Balkema.
Gallipoli, D., P. Grassl, S. J. Wheeler, and A. Gens. 2018. “On the choice of stress-strain variables for unsaturated soils and its effect on plastic flow.” Geomech. Energy Environ. 15 (Sep): 3–9. https://doi.org/10.1016/j.gete.2018.02.002.
Gallipoli, D., S. J. Wheeler, and M. Karstunen. 2003. “Modeling the variation of degree of saturation in a deformable unsaturated soil.” Géotechnique 53 (1): 105–112. https://doi.org/10.1680/geot.2003.53.1.105.
Gens, A. 1995. “Constitutive modelling: Application to compacted soil.” In Vol. 3 of Proc., of 1st Int. Conf. on Unsaturated Soils, Paris, 1179–1200. Rotterdam, Netherlands: A. A. Balkema.
Gens, A., M. Sánchez, and D. Sheng. 2006. “On constitutive modelling of unsaturated soils.” Acta Geotech. 1 (3): 137–147. https://doi.org/10.1007/s11440-006-0013-9.
Gray, W. G., and B. A. Schrefler. 2007. “Analysis of the solid phase stress tensor in multiphase porous media.” Int. J. Numer. Anal. Methods Geomech. 31 (4): 541–581. https://doi.org/10.1002/nag.541.
Houston, S. 2018. “It is time to use unsaturated soil mechanics in routine geotechnical engineering practice.” J. Geotech. Geoenviron. Eng., 145 (5): 02519001. https://doi.org/10.1061/(ASCE)GT.1943-5606.0002044.
Houston, S., and X. Zhang. 2021. “Review of expansive and collapsible soil volume change models within a unified elastoplastic framework.” Soils Rocks 44 (3): e2021064321. https://doi.org/10.28927/SR.2021.064321.
Houston, S., and X. Zhang. 2023. “A unified two independent stress variable approach to moisture-change-induced unsaturated soil volume change.” In Proc., 8th Int’l Conf. on Unsaturated Soils (UNSAT 2023). Section: Shrink-Swell & Collapse, E3S Web of Conf. V. 382 Number, 01001. London: International Society for Soil Mechanics and Geotechnical Engineering.
Jennings, J. E. 1960. “A revised effective stress law for use in the prediction of the behaviour of unsaturated soils.” In Pore pressure and suction in soils, 26–30. London: Butterworths.
Jennings, J. E. B., and J. B. Burland. 1962. “Limitations to the use of effective stresses in unsaturated soils.” Géotechnique 12 (Mar): 125–144. https://doi.org/10.1680/geot.1962.12.2.125.
Jommi, C. 2000. “Remarks on the constitutive modelling of unsaturated soils.” In Proc., Experimental Evidence and Theoretical Approaches in Unsaturated Soils; Proc., of an Int. Workshop, 139–153. Trento, Italy.
Khalili, N., F. Geiser, and G. E. Blight. 2004. “Effective stress in unsaturated soils: Review with new evidence.” Int. J. Geomech. 4 (2): 115–126. https://doi.org/10.1061/(ASCE)1532-3641(2004)4:2(115).
Khalili, N., and M. H. Khabbaz. 1998. “A unique relationship for x for the determination of shear strength of unsaturated soils.” Géotechnique 48 (5): 681–687. https://doi.org/10.1680/geot.1998.48.5.681.
Khalili, N., E. Romero, and F. A. Marinho. 2022. “State of the art report. Advances in unsaturated soil mechanics: Constitutive modelling, experimental investigation, and field instrumentation.” In Proc., ICSMGE 2022. Sydney, NSW, Australia: UNSW Sydney.
Kohgo, Y., M. Nakano, and T. Miyazaki. 1993. “Theoretical aspects of constitutive modelling for unsaturated soils.” Soil Mech. Found. Eng. 33 (4): 49–63. https://doi.org/10.3208/sandf1972.33.4_49.
Laloui, L., G. Klubertanz, and L. Vulliet. 2003. “Solid–liquid–air coupling in multiphase porous media.” Int. J. Numer. Anal. Methods Geomech. 27 (3): 183–206. https://doi.org/10.1002/nag.269.
Lloret-Cabot, M., M. Sánchez, and S. J. Wheeler. 2013. “Formulation of a three-dimensional constitutive model for unsaturated soils incorporating mechanical–Water retention couplings.” Int. J. Numer. Anal. Methods Geomech. 37 (17): 3008–3035. https://doi.org/10.1002/nag.2176.
Lloret-Cabot, M., S. J. Wheeler, and A. Gens. 2023. “Numerical integration of the Glasgow Coupled Model (GCM).” In Proc., E3S Web of Conferences 382, 15007, UNSAT 2023, Milos, Greece. Les Ulis, France: EDP Sciences. https://doi.org/10.1051/e3sconf/202338215007.
Lloret-Cabot, M., S. J. Wheeler, J. A. Pineda, D. Sheng, and A. Gens. 2014. “Relative performance of two unsaturated soil models using different constitutive variables.” Can. Geotech. J. 51 (12): 1423–1437. https://doi.org/10.1139/cgj-2013-0462.
Lloret-Cabot, M., S. J. Wheeler, and M. Sánchez. 2017. “A unified mechanical and retention model for saturated and unsaturated soil behaviour.” Acta Geotech. 12 (1): 1–21. https://doi.org/10.1007/s11440-016-0497-x.
Lu, N., J. W. Godt, and D. T. Wu. 2010. “A closed-form equation for effective stress in unsaturated soil.” Water Resour. Res. 46 (Mar): W05515. https://doi.org/10.1029/2009WR008646.
Lu, N., T. H. Kim, S. Sture, and W. L. Likos. 2009. “Tensile strength of unsaturated sand.” J. Eng. Mech. 135 (12): 1410–1419. https://doi.org/10.1061/(ASCE)EM.1943-7889.0000054.
Lu, N., and W. J. Likos. 2006. “Suction stress characteristic curve for unsaturated soil.” J. Geotech. Geoenviron. Eng. 132 (2): 131–142. https://doi.org/10.1061/(ASCE)1090-0241(2006)132:2(131).
Lu, N., B. L. Wu, and C. P. Tan. 2007. “Tensile strength characteristics of unsaturated sands.” J. Geotech. Geoenviron. Eng. 133 (2): 144–154. https://doi.org/10.1061/(ASCE)1090-0241(2007)133:2(144).
Matyas, E. L., and H. S. Radhakrishna. 1968. “Volume change characteristics of partially saturated soils.” Géotechnique 18 (4): 432–448. https://doi.org/10.1680/geot.1968.18.4.432.
Morgenstern, N. R. 1979. “Properties of compacted soils.” In Vol. 3 of Proc., 6th Pan-American Conf. on Soil Mechanics and Foundation Engineering: Contribution to the Panel Discussion Session IV, 349–354. Lima, Peru: Transport and Road Research Laboratory.
Nur, A., and J. D. Byerlee. 1971. “An exact effective stress law for elastic deformation of rock with fluids.” J. Geophys. Res. 76 (26): 6414–6419. https://doi.org/10.1029/JB076i026p06414.
Nuth, M., and L. Laloui. 2008. “Effective stress concept in unsaturated soils: Clarification and validation of a unified framework.” J. Numer. Anal. Methods Geomech. 32 (May): 771–801. https://doi.org/10.1002/nag.645.
Oberg, A., and G. Sallfors. 1995. “A rational approach of the determination of shear strength parameters of unsaturated soils.” In Proc., 1st Int. Conf. on Unsaturated Soils, Paris, 151–158. Rotterdam, Netherlands: A. A. Balkema.
Riad, B., and X. Zhang. 2020. “A modified state surface approach to study the unsaturated soil hysteresis behavior.” Transp. Res. Rec. 2674 (10): 484–498. https://doi.org/10.1177/0361198120937014.
Riad, B., and X. Zhang. 2022. “Fully characterizing the elastoplastic hydro-mechanical cyclic behavior of unsaturated soils using constant water content oedometer and direct shear tests.” Transp. Res. Rec. 2676 (10): 173–193. https://doi.org/10.1177/03611981221088775.
Romero, E., and C. Jommi. 2008. “An insight into the role of hydraulic history on the volume changes of anisotropic clayey soils.” Water Resour. Res. 44 (May): W12412. https://doi.org/10.1029/2007WR006558.
Roscoe, K. H., and J. B. Burland. 1968. “On the generalized stress-strain behavior of ‘wet’ clay.” In Engineering plasticity, edited by J. Heyman and F. A. Leckie, 535–609. Cambridge, UK: Cambridge University Press.
Schrefler B. A. 1984. “The finite element method in soil consolidation (with applications to surface subsidence).” Ph.D. thesis, Univ. College of Swansea.
Sheng, D. 2011. “Review of fundamental principles in modelling unsaturated soil behaviour.” Comput. Geotech. 38 (6): 757–776. https://doi.org/10.1016/j.compgeo.2011.05.002.
Sheng, D., D. G. Fredlund, and A. Gens. 2008. “A new modelling approach for unsaturated soils using independent stress variables.” Can. Geotech. J. 45 (4): 511–534. https://doi.org/10.1139/T07-112.
Sheng, D., S. W. Sloan, and A. Gens. 2004. “A constitutive model for unsaturated soils: Thermomechanical and computational aspects.” Comput. Mech. 33 (6): 453–465. https://doi.org/10.1007/s00466-003-0545-x.
Sivakumar, V. 1993. “A critical state framework for unsaturated soils.” Ph.D. thesis, Dept. of Civil and Structural Engineering, Univ. of Sheffield.
Sun, D. A., H. B. Cui, H. Matsuoka, and D. C. Sheng. 2007a. “A three dimensional elastoplastic model for unsaturated compacted soils with hydraulic hysteresis.” Soils Found. 47 (2): 253–264. https://doi.org/10.3208/sandf.47.253.
Sun, D. A., D. Sheng, and S. W. Sloan. 2007b. “Elastoplastic modelling of hydraulic and stress–strain behaviour of unsaturated soils.” Mech. Mater. 39 (3): 212–221. https://doi.org/10.1016/j.mechmat.2006.05.002.
Sun, D. A., D. Sheng, L. Xiang, and S. W. Sloan. 2008. “Elastoplastic prediction of hydro-mechanical behaviour of unsaturated soils under undrained conditions.” Comput. Geotech. 35 (6): 845–852. https://doi.org/10.1016/j.compgeo.2008.08.002.
Sun, D. A., D. C. Sheng, H. B. Cui, and S. W. Sloan. 2007c. “A density-dependent elastoplastic hydro-mechanical model for unsaturated compacted soils.” Int. J. Numer. Anal. Methods Geomech. 31 (11): 1257–1279. https://doi.org/10.1002/nag.579.
Tamagnini, R. 2004. “An extended Cam-clay model for unsaturated soils with hydraulic hysteresis.” Géotechnique 54 (3): 223–228. https://doi.org/10.1680/geot.2004.54.3.223.
Terzaghi, K. 1936. “Relation between soil mechanics and foundation engineering: Presidential address.” In Vol. 3 of Proc., 1st Int. Conf. on Soil Mechanics and Foundation Engineering, 13–18. Boston: Harvard Printing Office.
Toll, D. G. 2020. “Stress components in unsaturated soils.” Geotech. Eng. J of SEAGS & AGSSEA 51 (3): 19–25.
Vanapalli, S. K., D. G. Fredlund, D. E. Pufahl, and A. W. Clifton. 1996. “Model for the prediction of shear strength with respect to soil suction.” Can. Geotech. J. 33 (Mar): 379–392. https://doi.org/10.1139/t96-060.
Vaunat, J., E. Romero, and C. Jommi.2000. “An elastoplastic hydro-mechanical model for unsaturated soils.” In Experimental evidence and theoretic approaches in unsaturated soils, edited by A. Tarantino and C. Mancuso, 121–138. Rotterdam, Netherlands: Balkema.
Wheeler, S. J., and D. Karube. 1995. “State of the art report-constitutive modeling.” In Vol. 3 of Proc., of 1st Int. Conf. on Unsaturated Soils, Paris, 1323–1356. Rotterdam, Netherlands: A. A. Balkema.
Wheeler, S. J., R. S. Sharma, and M. S. R. Buisson. 2003. “Coupling of hydraulic hysteresis and stress–strain behaviour in unsaturated soils.” Géotechnique 53 (1): 41–54. https://doi.org/10.1680/geot.2003.53.1.41.
Wheeler, S. J., and V. Sivakumar. 1995. “An elastoplastic critical state framework for unsaturated soils.” Géotechnique 45 (1): 35–53. https://doi.org/10.1680/geot.1995.45.1.35.
Zakaria, I. 1995. “Yielding of unsaturated soil.” Ph.D. thesis, Dept. of Civil and Structural Engineering, Univ. of Sheffield.
Zhang, X. 2010. “Analytical solution of the Barcelona basic model.” Experimental and applied modeling of unsaturated soils, 96–103. Reston, VA: ASCE.
Zhang, X. 2016. “Limitations of the suction-controlled tests in the characterization of constitutive behavior of unsaturated soils.” Int. J. Numer. Anal. Methods Geomech. 40 (2): 269–296. https://doi.org/10.1002/nag.2401.
Zhang, X., J. Liu, and P. Li. 2010. “A new method to determine the shapes of yield curves for unsaturated soils.” J. Geotech. Geoenviron. Eng. 136 (1): 239–247. https://doi.org/10.1061/(ASCE)GT.1943-5606.0000196.
Zhang, X., and R. L. Lytton. 2009a. “A modified state surface approach on unsaturated soil behavior study (I) basic concept.” Can. Geotech. J. 46 (5): 536–552. https://doi.org/10.1139/T08-136.
Zhang, X., and R. L. Lytton. 2009b. “A modified state surface approach on unsaturated soil behavior study (II) general formulation.” Can. Geotech. J. 46 (5): 553–570. https://doi.org/10.1139/T08-137.
Zhang, X., and R. L. Lytton. 2012. “A modified state surface approach on unsaturated soil behavior study (III) modeling of coupled hydro-mechanical effect.” Can. Geotech. J. 49 (Jun): 98–120. https://doi.org/10.1139/t11-089.
Zhang, X., B. Riad, and E. Alonso. 2023. “Calibration of BBM parameters using the modified state surface approach.” In Proc., 8th Int. Conf. on Unsaturated Soils (UNSAT 2023). Physical, Numerical and Constitutive Modelling–Part II, E3S Web of Conf. V. 382. No.15001. Les Ulis, France: EDP Sciences. https://doi.org/10.1051/e3sconf/202338215001.
Zhang, X., and M. Xiao. 2013. “Using modified state surface approach to select parameter values in the Barcelona basic model.” Int. J. Numer. Anal. Methods Geomech. 37 (12): 1847–1866. https://doi.org/10.1002/nag.2112.
Information & Authors
Information
Published In
Copyright
This work is made available under the terms of the Creative Commons Attribution 4.0 International license, https://creativecommons.org/licenses/by/4.0/.
History
Received: Nov 3, 2023
Accepted: Mar 18, 2024
Published online: Jun 4, 2024
Published in print: Aug 1, 2024
Discussion open until: Nov 4, 2024
ASCE Technical Topics:
- Effective stress
- Engineering fundamentals
- Geomechanics
- Geotechnical engineering
- Material mechanics
- Material properties
- Materials engineering
- Measurement (by type)
- Saturated soils
- Shear strength
- Soft soils
- Soil mechanics
- Soil properties
- Soil strength
- Soil stress
- Soils (by type)
- Strength of materials
- Stress (by type)
- Structural analysis
- Structural engineering
- Unsaturated soils
- Volume change
- Volume measurement
Authors
Metrics & Citations
Metrics
Citations
Download citation
If you have the appropriate software installed, you can download article citation data to the citation manager of your choice. Simply select your manager software from the list below and click Download.