Abstract
Biogeotechnical research has inspired revolutionary ideas to claim the status of a distinctive branch of geotechnical engineering. Most notably, underground carbonate mineralization, most commonly referred to as microbially induced carbonate precipitation (MICP), has been seen as a promising solution to mitigate multiple problems, most notably increasing the bearing capacity of soils, slope stabilization, liquefaction mitigation, and erosion protection. To date, it is fair to say that MICP has moved past the proof-of-concept phase and is growing in terms of technology readiness. In this work, a comprehensive review is provided, ranging from MICP’s core fundamentals to recent breakthroughs and milestones, specifically targeting three core axes, namely, the achieved mechanical performance, modeling approaches, and upscaling considerations. From the return of experience in over two decades of research and considering the most recent opportunities and challenges, this review covers more than 150 articles published after 2019, introduces a synthesis of the trends in mechanical performance captured for biocemented materials, and concludes with a holistic overview of the future prospects of biocementation.
Biogeotechnical Engineering
With a continually rising demand, constrained by limited available resources, geotechnical works have been increasingly under pressure to evolve and combine technical innovation, economic efficiency, and environmental responsibility. New or existing infrastructures have been pushed to new limits, while their foundations require retrofitting to keep up with increased utilization or rehabilitation loads. An increasing number of people settle in natural disaster–prone regions exposed to high risks of landslides and rising sea levels or earthquakes, resulting in a soil stabilization market forecasted to reach US$33.3 billion by 2025 (IndustryARC 2021). In parallel, ecological concerns are challenging the status quo of traditional geotechnics in favor of ecofriendly alternatives. Instead of traditionally relying on overdesigned or energy-intensive solutions, biogeotechnical engineering is promoting a change in paradigm, preferring interventions that are less disruptive to underground ecosystems. In this context, the natural environment is then seen as a viable resource to protect, a source of inspiration to mimic, and a construction asset to mobilize (Suer et al. 2009; DeJong et al. 2010a, b, 2013; Terzis and Laloui 2019a).
Biomediation primarily seeks to identify naturally occurring biochemical processes, which can then be accelerated in time or relocated in space, altering the initial soil state to improve its performance (Dejong and Kavazanjian 2019). At its core lies a change in paradigm, no longer considering soil as an inert phase diagram solely formed of minerals and pore fluids but rather as an interactive living ecosystem capable of modifying its rheological characteristics to external stimuli. With more than 109 microorganisms found in a cubic centimeter of soil near the surface (Mitchell and Santamarina 2005; Nature Reviews 2011), the underground could be auspicious to a stimulation of targeted indigenous microorganisms or to receive an augmentation of exogeneous species. Generally, three principal biomediations can be differentiated based on the main resulting by-product of the chemical relations taking place, namely, biomineralization (inorganic precipitate), biofilm (organic precipitate), and biogas (gas generation). Biofilms most generally include an accumulation of cell colonies, extracellular polymeric substances, and diverse other molecules or retained particles (Fatehi et al. 2021). Biofilms can considerably reduce the hydraulic conductivity of soils (Proto et al. 2016; Hata 2019; Zhang et al. 2021b), or even strengthen the soil by impacting its suction stress characteristics (Shariq et al. 2021). They are mainly considered for immediate interventions, such as emergency stabilization of pollutants or dewatering around excavations, due to their short-term and reversible effect. Bio-gas-induced desaturation of soils is possible through bacterial-induced biogenic gas emission, the most notable being nitrogen via denitrification, due to its low solubility in water (O’Donnell et al. 2017). Above all, this approach is promising as a liquefaction mitigation mechanism (He et al. 2013; O’Donnell et al. 2019a). Last, ex vivo biomineralization, most notably through microbially induced calcium carbonate precipitation (MICP), results in the formation of crystals in the surrounding environment of microorganisms serving as nucleation sites (Terzis and Laloui 2019a). As such, the precipitated calcium carbonate serves as a bonding agent within the soil skeleton, conveying the name biocementation to the process. Among these notable biomediated techniques, biomineralization through MICP has received the most attention from both academia and industry as the most promising soil improvement technique. This success is partially due to its nature as a research topic soliciting multidisciplinary interest at the confluence of microbiology, geochemistry, and civil engineering but also implies different expectations regarding its foreseen applications.
The work herein is focused on biomineralization, covering a review of its breakthroughs and challenges relative to the fundamental biochemical process of MICP. Central aspects governing the current state and future of biocementation as a soil improvement technique are then covered in respective sections, from mechanics to modeling and upscaling considerations, before finally addressing the new frontline and directions toward mainstream applications.
Microbially Induced Carbonate Precipitation
Biochemical and Microscopic Insights
Microorganisms hold a central role in induced calcium carbonate precipitation, namely, in their capacity to (1) generate carbonate ions through one or more bioprocesses, (2) catalyze the latter to produce a significant amount of carbonate in an engineeringly relevant time frame, (3) shift the local alkalinity in favor of carbonate equilibrium, and finally, (4) provide nucleation sites during precipitation. Driving the formation of carbonate is possible through multiple metabolic pathways, such as ureolysis, denitrification, sulfate reduction, iron reduction, and ammonification, of which ureolysis was seen to yield faster and more efficient carbonate alkalinity by multiple orders of magnitude (Graddy et al. 2018, 2021). Ureolytic MICP utilizes a urease-producing microorganism to catalyze the hydrolysis of urea into ammonia and carbonic acid, as per Eq. (1). Under neutral pH conditions, an aqueous ammonia equilibrium reaction then follows, producing ammonium and hydroxide ions in solution, as in Eq. (2), resulting in a more alkaline environment. Consequently, the carbonate equilibrium of Eq. (3) shifts in favor of bicarbonate and carbonate formation. When coupled with a supplied calcium source, as in Eq. (4), carbonate and calcium ions form solid calcium carbonate in a supersaturated system.Traditionally, most studies have used Sporosarcina pasteurii as the urease-producing microorganism, which is nonpathogenic, easy to grow, and exhibits sufficient resilience to different environmental conditions. It can further attach to grain surfaces and yields a high urease activity rate and a predominance of precipitates in stable calcite phase (Harkes et al. 2010; Mortensen et al. 2011; Omoregie et al. 2017; Jain and Arnepalli 2020; Marzin et al. 2020; Tang et al. 2020). More recently, other works have utilized other strains to explore alternatives that may have different physiologies, such as shapes (cocci or bacillus), surface textures (with or without fimbriae), growth conditions (pH and temperature), or treatment configurations (augmentation and stimulation) (Hata et al. 2020; Riveros and Sadrekarimi 2020a; Almajed et al. 2021; Graddy et al. 2021).
(1)
(2)
(3)
(4)
While one of the most abundant constituents within geomaterials, CaCO3 produced through MICP may not hold the same characteristics and thus the same properties as its geological mineral counterpart. First, depending on surrounding environmental conditions (such as reactants concentrations, temperature, pressure, bacteria strain, urease activity, nucleation sites, etc.), biogenically induced CaCO3 can assume and potentially retain different phases, starting from amorphous to vaterite spherulites, needle-shaped particles of aragonite, and rhombohedral calcite (Clarà Saracho et al. 2020b; Zambare et al. 2020). Among these different phases, the latter is the preferred form in terms of polymorph stability, solubility, and mechanical performance in soil due to its capacity to attach to surfaces, envelop contact points, and fill pore spaces (Zambare et al. 2020; Murugan et al. 2021; Xiao et al. 2021a; Zhang et al. 2021a). Insights into the real morphologies of CaCO3 are captured by scanning electron microscopy (SEM) in Fig. 1. A closer inspection in Fig. 2 of the calcite also reveals the presence of flaws as a result of hierarchal crystal growth and gradual layered deposition, in which traces of encapsulated bacteria can also be observed amid larger homogeneous crystals, as shown in Fig. 2(a), or in contrast, exhibits the inclusion of voids in between individually deposited crystals of different sizes, as seen in Fig. 2(b). Such microscale features could directly alter the load-displacement curves, reducing the elastic modulus by 9.9% and up to 24.8% during nanoindentation tests (Huang et al. 2021b). More generally, the dependence of the phase transition and nanomechanical properties of precipitated crystals on environmental conditions opens the possibility for tailor-made alternative cementing materials. In particular, studies targeting different microorganisms, enzymes, and carbon sources found that lower urease activity favored larger average calcite crystals and higher nanoindentation moduli (Dhami et al. 2016; Heveran et al. 2019).
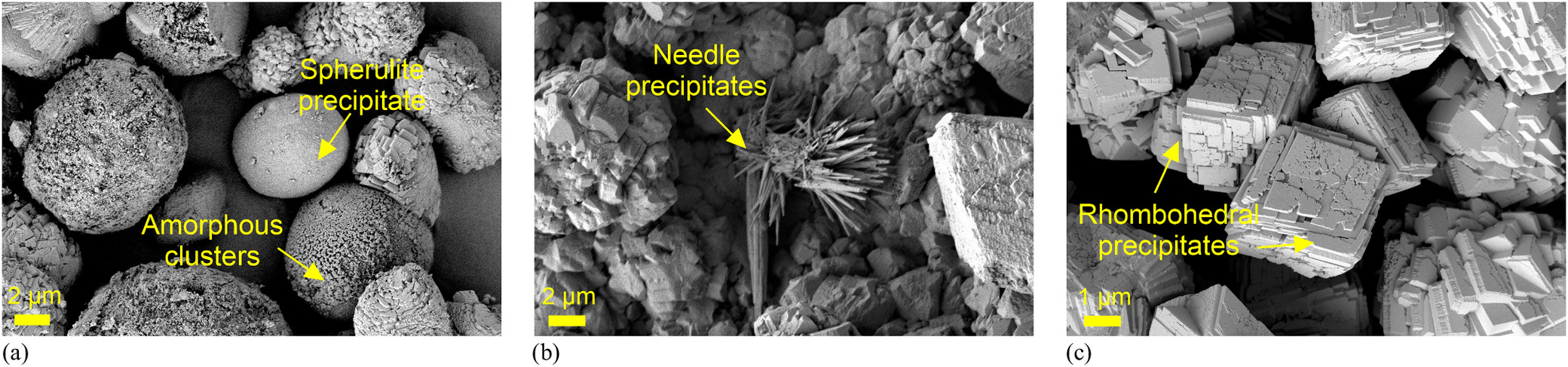

In addition to its morphology, the location of CaCO3 deposition is crucial in governing its impact on the mechanical response in soil. Hence, it is possible to differentiate between active and passive CaCO3 regarding its role in the transmission of intergranular forces in the soil skeleton. Fig. 2(c) shows the configuration of contact or matrix supporting bonds in contrast to the passive deposits coating the grain surface, only contributing to the densification or interlocking of particles during shear. In addition to biohydrochemical factors affecting the performance of MICP (Tang et al. 2020; Xu et al. 2021), intrinsic soil properties such as granulometry and mineralogy and initial state variables such as compacity or saturation level have also been shown to influence the efficiency of a treatment on soil performance. This is caused by shifting the deposition in favor of active CaCO3 by preseeded growth, for instance, due to an initially larger number of contact points between grains, or by localizing biochemical reactions in the menisci, for the case of a dense or partially saturated soil, respectively (Haouzi et al. 2018; Mahawish et al. 2018; Xiao et al. 2019a; Cheng et al. 2020; Wang et al. 2021b). Even the particle shapes, aspect ratio, surface texture, and pore-water composition (e.g., fresh versus seawater) were shown to affect the mineralogy, morphology, and reaction kinetics of ureolytic biocementation (Nafisi et al. 2018; Xiao et al. 2019c; Burdalski et al. 2022).
Advances in X-ray computed tomography (XRCT) opened the possibility for finer qualification and quantification of microscopic features that support the findings generally obtained at the macroscale level. From individual slices to a complete 3D reconstruction of the solid matrix, XRCT analyses have been shown to adequately capture the cementation level as well as changes in the lattice properties following a treatment, namely, the evolution of dry density, void ratio, and porosity, which exhibit excellent agreement with laboratory measurements (Mahawish et al. 2019). In addition to different microstructural quantifications, determining the orientation of particles, including that of calcite bonds and their average diameter, was also possible through XRCT, even identifying that 75% of total bonds were active (Terzis and Laloui 2018, 2019b). Similar findings were reported by Dadda et al. (2019b), in which XRCT captured 80% of calcite precipitating at the contacts between grains. Furthermore, the study investigated the evolution of multiple microstructural properties with the volumetric fraction of calcite, such as contact numbers, surface, orientation, and especially contact type, differentiated between frictional (3% content threshold), mixed, or cemented contacts. Such microstructural parameters are key for analytic models and could eventually be used as inputs to estimate the evolution of the elastic moduli and cohesion with cementation content (Dadda et al. 2019a). In this optic, more recent studies could even quantify micromechanical parameters relative to tensile, shear, fracture, and cyclic shear strength of CaCO3 bonds between glass beads, opening the door toward direct calibration of bonding parameters for many modeling applications (Ham et al. 2022; Gao et al. 2023; Xiao et al. 2023).
Micro-based frameworks also attracted renewed interest for the study of MICP processes using microfluidic chips, which, coupled with optical microscopes and XRCT, allow a direct spatial and temporal characterization of MICP. Hence, the homogeneity of bacterial distribution, adhesion rates with different ionic strengths (Marzin et al. 2020), and time-dependent crystal growth under continuous or sequential injections were monitored (Wang et al. 2019a, b). In more recent studies making use of state-of-the-art microfluidics, intrinsic properties and the architecture of porous networks along with the chemical concentration gradients were seen to have direct influence on the distribution, shape, and size of CaCO3 deposits (Elmaloglou et al. 2022; Xiao et al. 2022a). Microfluidics also allowed the testing of multiple variables such as treatment scheme (mixing, drop-based), bacterial strains, kinetics, encapsulation, permeability, and tortuosity for MICP and for enzymatically induced carbonate precipitation (EICP) (Kim et al. 2020; Zambare et al. 2020; Wang et al. 2021e; Weinhardt et al. 2021; Xiao et al. 2021a; Zehner et al. 2021).
New Horizons
Beyond conventional MICP, new frontiers for the technology mainly include thermal or electrical considerations and the pairing of MICP with additives. Indeed, in addition to making multiple improvements in the mechanical properties, MICP was observed to alter the thermal characteristics of soils. Using a dual-probe heat-pulse sensor, recent studies have captured an increase in thermal conductivity of soils with increasing number of treatment cycles, in addition to its variability at the saturation level (Ding et al. 2019; Wang et al. 2020b). It was concluded that the potential of using MICP to enhance the heat transfer efficiency in sands is the highest under dry conditions or at low saturation degrees. Similar results were found by Martinez et al. (2019), in which the enhancement following biocementation varied between 330% and 15% for dry and saturated states, respectively, traditionally attributed to the formation of thermal bridges among soil particles (Venuleo et al. 2016). As such, MICP is proposed to enhance the efficiency of energy piles and ground source heat pump systems, especially targeting their limitation in dry soils, thereby increasing the possibility of expanding geothermal applications to arid environments (Wang et al. 2020b). Xiao et al. (2021b) also investigated the effect of gradation, void ratio, and treatment level on the thermal conductivity and found the greatest improvement for an initial degree of saturation between 0.82 and 0.85. Alternatively, thermal conductivity could serve as an indicator of cementation level and contact quality since its evolution with treatment resembled that of shear-wave velocity (Martinez et al. 2019).
From another perspective, subjecting the targeted soil volume to an electric field during treatment can make use of multiphysical phenomena, such as electrostatics or electrokinetics, that hold promising enhancements for electrically assisted MICP (EA-MICP) compared with a regular treatment. Electrostatics, for instance, can induce a polarization of grain surfaces, hence favoring the attachment of bacteria and precipitated crystals, while alternatively, different electrokinetic flows can take place. More precisely, electroosmosis, electromigration, and electrophoresis correspond to the movement of liquid, ions, and particles, respectively. As such, recent studies have traditionally focused on EA-MICP as a way to enhance the transport and distribution of solutions, reactants, and microorganisms to amplify the impact of regular biocementation when addressing geotechnical challenges or in removing undesired by-products. Most notably, EA-MICP can be used to treat fine soils. For instance, during electroosmotic consolidation of marine clays (Tian et al. 2021), the treatment significantly reduced uneven settlement, while increasing shear strength. Other typically sidelined soils with regard to traditional MICP can also be accessed through electric stimulation. For example, organic soils were successfully treated using both premixing and electrokinetic injections (Safdar et al. 2021), and the fractal pore characteristics of natural aeolian fine sand were studied with different electrifying times (Li et al. 2021a). In a recent work, the effect of electric currents of different forces and polarities on calcium carbonate precipitation were assessed under various chemical conditions (Terzis et al. 2020a), revealing a detrimental impact of EA-MICP with calcium chloride due to hypochlorination, but enhanced crystals of larger sizes and growth directionality when using alternative calcium sources. A recent study even applied solar-powered electrobiogrouting to increase the compressive strength of a soil, while simultaneously minimizing its contamination by retaining the produced ammonium in the cathode chamber by a graphite electrode (Keykha and Asadi 2017).
It is also possible to resort to the addition of external additives, mainly to expand the technique toward more problematic soils. In a recent study, while a regular treatment (without additives) barely altered the unconfined compressive strength (UCS) of amorphous peat, it was possible to reach a sixfold improvement level through the addition of a ground calcite powder from scallop shells, which generated additional nucleation sites and provided an inner mineral skeleton connecting the precipitated CaCO3 bridges (Gowthaman et al. 2021). Targeting a similar peat soil, in another investigation, an 80-fold improvement in undrained shear strength was reported when adopting a fiber-incorporated biocementation treatment (Chen et al. 2021). Another emerging MICP admixture is a clay additive such as bentonite, which can be mixed and suspended in a bacterial solution that is administered as part of the treatment. Bentonite-assisted MICP, especially for coarse soils, was seen to increase the bacterial activity retention, produce a larger amount of precipitated CaCO3, exponentially decrease the permeability, and substantially improve the mechanical response (Ma et al. 2021; Zhao et al. 2021). Alternatively, MICP could be deployed with surface treatments to delay crack initiation and remediate the desiccation cracking of bentonite or clayey soils (Zhu et al. 2019) or to rehabilitate deteriorated cement-treated soils by recrystallization (Hata et al. 2018). Finally, some studies have attempted to bypass the need for microorganisms or enzymes for urea hydrolysis in MICP and EICP in favor of chemically induced carbonate precipitation (CICP) by supplying direct CO2 gas with calcium hydroxide solution without needing or generating other reactants or undesired by-products (Park et al. 2020). Eventually, more elaborate uses of MICP were attained, including the fabrication of capsules (Clarà Saracho et al. 2021), 3D soil structure printing (Nething et al. 2020), and even extraterrestrial applications, with current tests targeting lunar or Martian soil simulants, including modifications to traditional MICP bioprocesses to consider a Mars-compatible atmosphere (Gleaton et al. 2019; Dikshit et al. 2021; Naser and Chen 2021).
Mechanics of Biocemented Soils
Shear Behaviors
Addressing how MICP alters the shear response of a treated geomaterial has been the subject of several studies ever since its first consideration as a soil improvement process. In unconfined crushing, the range of reported strength increased with cementation contents following different trends, as compiled in Terzis and Laloui (2019a). Proposing direct correlations between treatment variables and improved mechanical responses, however, remains limited, due to the multileveled dependence of the final improvement on the intrinsic material properties as well as the treatment conditions. As such, many proposed correlations rely on simplifications. More recent works have attempted to bridge this gap by incorporating more influencing factors in the proposed correlations or lines of analysis, for instance by making use of the porosity to cement ratio when dealing with deformability or strength improvements and correlations (Harran et al. 2022; Xiao et al. 2022b).
In contrast, it is overall well accepted that the confined response is characterized by an increased stiffness, accompanied by a distinctive peak strength, followed by a pronounced softening, with increased dilative tendencies. While some exponential trends were noted for the increase in UCS with cementation content (Konstantinou et al. 2021), studies shifted to a finer understanding of the complexity of confined behaviors and their predictability with soil- and treatment-related variables.
Using drained triaxial tests for different sands, cementation, and confinement levels, Nafisi et al. (2020b) differentiated a strain-hardening response for lightly cemented specimens, while capturing the most pronounced impact of MICP (17 times higher shear strength, peak and softening) at higher contents and under lower confining stresses compared with the untreated state. Compared with a bilinear or a nonlinear envelope, the linear case was seen to overestimate the strength at low confining stresses, for which the cohesion intercept (c′) and angle of internal shear resistance (φ′) could still increase by more than 100 kPa and 7.7°, respectively. Focusing on calcareous sands, Liu et al. (2019) reported an exponential increase in c′, while φ′ remained essentially constant. In line with what was reported, another study captured the impact of a treatment to be essentially translated by a continuous increase in c′ (from 0 to 530 kPa) yet characterized by a capped improvement with respect to φ′ (from 32° to 45°) that did not improve further for CaCO3 contents as high as 14% (Wu et al. 2021). The work also examined the volumetric response, reporting more dilation with MICP treatment, even when the natural state at the same density was still exhibiting contractive tendencies Wang et al. (2021a) established a systematic comparison between biocemented and portland-cemented samples for various treatment and confinement levels. They reported an increased brittleness in the macroscopic response conveyed by the CaCO3 bonds, marked by a similar higher increase in stress ratio with the dilatancy ratio for biocementation compared with artificial cementation. From a complementary perspective, Nafisi et al. (2021) investigated the response following different stress paths, namely, in axisymmetric compression, radial extension, constant mean effective stress, and constant deviatoric stress, while varying the base sand and treatment levels. Failures were observed at increasing strains and peak values for the different stress paths, namely, radial extension (100 kPa), constant deviatoric stress (200 kPa), constant mean effective stress (300 kPa), and axisymmetric compression (860 kPa) cases. Concurring results were reported using CICP, for which φ′ increased between 6° and 17°, while the improvement in c′ remained constant at approximately 39–46 kPa for CaCO3 contents in the range of 2%–20% (Park et al. 2020). Likewise, EICP yielded similar results to those reported for MICP at even lower cement contents (Hoang et al. 2019; Nafisi et al. 2019). A summary of the reported increase in peak φ′ and c′ in recent works is given in Fig. 3, including some select older references (Cheng et al. 2013; Feng and Montoya 2016). Overall, the increase in strength parameters with CaCO3 content is evident, yet diverges in its main contributors between c′ and φ′ across studies. For instance, some reported a clear enhancement of both parameters, while others suggested a predominant increase in one, while the other was largely unaffected or stagnant with increasing amounts of biocement. In addition, the choice of the base geomaterial, granulometry, and compacity evidently plays a crucial role in the level of expected improvement in the mechanical response, as reported for a large set of geomaterials with the same calcite content. A finer understanding of the contribution of the geomaterial properties is therefore necessary (Tang et al. 2020) as they seem, in addition to the often-considered treatment scheme and the saturation level, to hold a central role on the expected enhancement or the failure envelope and its fitted parameters. Choi et al. (2020) provided additional data and correlations relative to the UCS and Mohr–Coulomb parameters.

While the critical state is traditionally considered unaffected by biocementation (Gai and Sánchez 2019; Gajo et al. 2019), older propositions (DeJong et al. 2010a) and more recent experimental works (Wang et al. 2021a) seem to suggest some form of impact, even when shearing under constant volume is reached. Riveros and Sadrekarimi (2020a) reported no impact of the treatment on the critical state friction angle , in contrast to the noticeable increase in the strength parameters. However, the precipitated mass of CaCO3 and the crushing-induced compressibility reportedly resulted in a shift in the critical state line toward a denser and steeper (lower void ratios) state. A finer understanding of the exact impact that MICP can have on the critical state, also necessitates physical and microstructural investigations, beyond the dependence on mathematical fits for the choice of failure envelopes.
Beyond stress–strain-dilation frameworks, the shear response of biocemented soils was addressed in wider optics, relative to the pullout or interface shear, the erosion or scouring resistance, and more. Experimental results using MICP to treat a calcareous sand–steel interface revealed a net increase in the peak shear strength, along with a reduction in shear displacement and more dilation (Li et al. 2021c), mainly captured through an increase in the cohesion intercept along the Coulomb criterion. Alternatively, MICP could be coupled with a geosynthetic of different configurations, enhancing the pullout resistance by 13%–38% for only a 0.65% CaCO3 content (Gao et al. 2021). Relative to both shearing and erosion, through a submerged impinging jet test, MICP was observed to increase the critical shear stress (at which no more scour occurs under the constant pressure), while decreasing the erosion rate by three and four orders of magnitude, respectively (Do et al. 2019). Jiang and Soga (2019) differentiated the erosion resistance for soils with a gravel- or sand-supported structure, revealing a better compatibility with the former, while Clarà Saracho et al. (2020a) identified block erosion and crack formation as the main erosion mechanisms for the treated state. Another study similarly focused on enhancing the hydraulic and erosional behavior of internally unstable and poorly graded soils (Haouzi et al. 2020). With respect to traditional foundations, biogrouting around a precast pile resulted in a fourfold increase in its bearing capacity, while identifying the main source of improvement as the toe-bearing resistance, which reportedly showed a ninefold increase (Xiao et al. 2020b).
Some recent works combined the mechanics of biocemented soils with less conventional physical aspects. For instance, it was possible to monitor the collapse mechanics under uniaxial stress by means of crackling noise (Wang et al. 2021c), capturing that within a single MICP-treated sample, some parts behaved exactly like a regular sand in between biocement structured regions. From another side, the mechanical response of a temperature-controlled one-phase MICP was examined by means of triaxial tests (Xiao et al. 2021c). MICP was even seen to alter the water retention properties of an unsaturated sand and a low-plastic clay (Saffari et al. 2019).
Deformability Characteristics
While extensive studies have focused on the large-strain and failure states of biocemented soils, fewer investigations have focused on their deformability under representative conditions relative to many foreseen geotechnical applications, such as in the prepeak phase under serviceable loads or in constrained k0 configurations.
Recently, Li et al. (2021b) captured a change from high to low compressibility of an expansive soil for treatment contents ranging between 2% and 8%, while the preconsolidation pressure almost doubled with the treatment (from 43 to 74 kPa). Xiao et al. (2020a, c) investigated the impact of very high stresses in oedometric compression by applying loads as high as 30 MPa. MICP was observed to restrain sand particle breakage, while distinguishing between phases of bond breakage and grain fracture. Similar results were reported for lower applied stresses reaching 1,000 kPa by Harran et al. (2022), capturing an increase in the preconsolidation pressure and oedometric modulus, while decreasing the compression (Cc) and recompression (Cr) indices with the treatment of two types of sand. The work also captured the increase in delayed deformation with time under sustained loading, not due to a conveyed ductility of the precipitated CaCO3 but rather a shift in the “rearrangement potential” of grains to be released after bond breakage occurs. Such findings are in line with the larger proportion of time-dependent deformation of coral compared with silica sands (Wang et al. 2020a). Concurring results were proposed under pseudo-k0 loading conditions, in which MICP reduced Cc, Cr, and the coefficient of volume compressibility (mv) by approximately 40%–50% (Arboleda-Monsalve et al. 2019). Additionally, the study reported a rather insensitive response to different strain and stress rates, reinforcing the bond-degrading dependence rather than the viscous behavior of a treated soil lattice. By means of a physical scaled model, MICP-reinforced stone columns in soft clays were seen to decrease both axial strains and bulging measured in radial strains by averages of 50% and 70%, respectively (Mahawish et al. 2021). Beyond traditional geomaterials, Montoya et al. (2019) successfully addressed the remediation of the excessive compressibility of coal ash using MICP, with similar trends captured for Cc and mv as those noted in previous studies in granular materials. In contrast to the compressibility response, MICP can also hold the potential to mitigate the swelling of expansive soils, in which for instance, biocementation reportedly reduced the swelling pressure (from 41 to 15 kPa) and shifted a high-plasticity (CH) clay to a low-plasticity (CL) index (Tiwari et al. 2021).
Some findings of the main covered works targeting the deformability aspects are summarized in Fig. 4, in addition to selected older studies (Lee et al. 2013; Feng and Montoya 2014; Lin et al. 2016); these findings express the difference in axial strain reduction between each biocemented soil sample and the untreated equivalent. The results consistently show a high compressibility reduction for low stresses, which then degraded at different rates with increasing axial load, depending on the initial geomaterial, treatment level, and efficiency. Even at a high vertical effective stress of 1,000 kPa, many of the reported data sets in Fig. 4 still exhibit a more than 40% reduction in accumulated axial stress. This finding highlights the effectiveness of the technique when adequate treatment is deployed in conjunction with the stress levels normally encountered in the field within various applications at shallow depths. Similarly to what was noted in the previous section, the type of geomaterial, granulometry, and initial density hold a significant impact comparable to that of the actual biocement content on the improvement and degradation of the improved mechanical response. Overall, the results of most studies are in agreement with the fact that the response for treated soils remains soil-like, in which degradation of soil structure takes place progressively with increasing stress without immediate collapse (Feng and Montoya 2014; Harran et al. 2022; Li et al. 2021b; Montoya et al. 2019; Xiao et al. 2020a, c).

Tensile Strength
Fewer studies have targeted the tensile strength of soils, traditionally considered quasi-negligible compared with that of other geomaterials such as rocks. This is, however, not the case for naturally or artificially cemented soils, whose tensile behavior remains understudied. In the laboratory, tensile strength characterization can be achieved through different tests, the most notable being (1) Brazilian split tensile strength (STS), (2) uniaxial, and (3) bending tensile tests, or even within triaxial conditions (Péron 2013).
In recent studies by Sharma et al. (2021a, b), the rock-like behavior of a poorly graded liquefiable sand was characterized, among other aspects, in split tension after being subjected to various biotreatment schemes. The highest recorded STS was 437 kPa for S. pasteurii (compared with B. sphaericus) for an average calcite content of 12%. The permanence of biocemented sand to retain this improvement was also assessed, with less than a 10% reduction in tensile strength noted after 10 freeze–thaw cycles in all biotreated samples. Using a surface percolation treatment technique, another study yielded comparable results with a maximum STS of 300 kPa, which then rapidly decreased with distance from the sample surface (Karimian et al. 2021). In another investigation, Ahenkorah et al. (2020) captured higher STS values exceeding 1 MPa, particularly for EICP, which showed a larger improvement than MICP at the same CaCO3 content. When tested in direct tension, Nafisi et al. (2020a) reported a lower tensile strength for fine sand than for medium and coarse sands, while the results overall fluctuated between 212 and 712 kPa.
In other studies, both the effect of fibers and MICP treatment were combined to maximize the enhancement of the tensile strength. The reported STS values of fiber-reinforced MICP cemented loose sand typically vary between 0.3 and 2.2 kPa (Fang et al. 2020; Zhao et al. 2020), while increasing the ductility of the response. By using natural wool instead of synthetic fibers, Yao et al. (2021) achieved a similar STS and flexural strength between 0.2 and 1.1 MPa. Another alternative is the use of basalt fibers, as covered in the work of Xiao et al. (2019b), yielding a tensile strength of 1.1 MPa. In addition to the characterization of the peak value and the stress–strain responses, a splitting secant elastic modulus could be measured, for instance, varying between 5.0 and 7.5 MPa (for STS 48.1–88.5 kPa) or even reaching 18.8 and 25.9 MPa (for higher STS values of 230–592 kPa) (Choi et al. 2016, 2019). Aside from typical tensile strength improvement, Cardoso et al. (2021) assessed the joint sealing capacity of MICP between basalt stone disks, whose maximum tensile strength could reach 5.67 MPa or half the values measured for the intact rock (8 MPa). In comparison, portland cement–treated soils at contents ranging between 4% and 10% exhibit comparable tensile strength with the biocemented cases. More precisely, in a Brazilian setup, strength values of 140–300 kPa were recorded (Das et al. 1995) and ranged from 100 to 500 kPa in a direct tension configuration (Consoli et al. 2010; Namikawa et al. 2017).
A comprehensive summary of eight recent studies on the tensile strength of biocemented soils is shown in Fig. 5. Due to the friable nature of low-treated specimens, tensile experiments usually require higher minimum calcium carbonate contents, with practically no data points below 4% and eventually reaching values as high as a 30%. Overall, the tensile strength values increased steadily with cementation level, although at different rates between studies. These variabilities, in addition to some scatter within the same experiments, are due to the variable geomaterials, bacterial strains, and treatment schemes used. Additionally, the addition of fibers of different types (natural or synthetic) and percentages further contribute to the divergence observed between some studies. Further characterization of the tensile stress–strain paths of biocemented soils may well target the impact of confinement for lower cementation amounts, with and without fibers, since in situ treatment levels exceeding 10% are not always pragmatic.

Cyclic Response
Beyond monotonic behaviors, the cyclic response of biocemented soils has been predominantly studied with respect to liquefaction. To this end, two types of approaches can be identified, with experiments that (1) aim to reproduce earthquake analogous conditions through the use of shake tables, possibly in combination with centrifuge tests, or (2) rely on traditional setups, such as triaxial or direct simple shear (DSS), to impose undrained cyclic stress paths.
Using centrifuge shaking models, MICP treatment improves the soil behavior under dynamic loading, in addition to reducing excess pore pressure generation and settlement (Montoya et al. 2013). More recent studies tend to consolidate such findings and expand them to include more diverse considerations. A recent study, using an instrumented 70 × 65 × 46 cm physical model, captured various improvements in calcareous sand (Zhang et al. 2020), in which the treatment resulted in a maximum pore pressure ratio never exceeding 0.7 (instead of 1 to reach liquefaction). This ratio was reached as late as 20 s after shaking (compared with 3 s for untreated), while dynamic stress–strain paths still captured some residual strength even after 30 s of shaking. In another setup of comparable dimensions mounted on a 1 m centrifuge, a peak base acceleration of 0.45g was needed to liquefy an MICP-treated sand (2% CaCO3 content) instead of 0.12g for the natural state (Darby et al. 2019). Similar results were reported in a centrifuge study for an MICP-treated zone (Huang et al. 2021a). Notably, some shake table studies, however, report an increased surface acceleration following an MICP treatment (Montoya et al. 2013; Darby et al. 2019; Zhang et al. 2020), potentially suggesting the need for a holistic site response evaluation with ground treatment.
Undrained conditions allow the direct application of a controlled number of cycles, converging toward complementary results to shake table experiments. In undrained triaxial testing, Xiao et al. (2018) captured an increase in the number of cycles to reach liquefaction (NL) from 31 for untreated to 72, 118, and 135 for treatments of 0.2, 0.4, and 0.6 L of cementation solution, respectively, in addition to a reduction in the accumulated axial strains. In follow-up work using similar calcareous sand, cyclic experiments showed that MICP could change the liquefaction failure mechanism from flow failure to cyclic mobility, while also significantly affecting the excess pore pressure generation response of initially loose specimens (Xiao et al. 2019a). Specifically, biocementation was shown to exhibit greater efficiency in improving the cyclic resistance than pure densification of the same natural state at higher relative densities. Comparable results could be found when using EICP on two different sands, in which the treatment level required to reach a given liquefaction resistance could be significantly reduced by decreasing the degree of saturation during curing (Simatupang et al. 2018). In parallel, other studies have relied on a direct simple shear framework for their cyclic campaigns. For instance, Zamani and Montoya (2019) varied the fines content in silty sand with MICP treatment, capturing a 0.1–0.3 increase in the cyclic resistance ratio (CRR); the best improvement was recorded at 35% fines (CRR = 0.41) due to their beneficial impact on the relative density and fabric structure. In another work by Riveros and Sadrekarimi (2020b), it was found that MICP treatment increased NL from 14 to 94. More particularly, when comparing liquefaction at 15 cycles, MICP-treated samples exhibited cyclic resistances up to 67% higher than those of the untreated sand, surpassing the improvement achieved by solely increasing the relative density. Additionally, using DSS, it was shown that even very low treatment levels (a content of 0.1% to 1%) could significantly alter the cyclic resistance of loose sands by increasing NL (Lee et al. 2020), for instance, from 30 (untreated) to more than 9,000. Cross comparisons across studies are usually limited by the divergence in the adopted experimental conditions for each case. Recently, a cyclic improvement factor (If) has been more frequently reported, defined as the ratio of the cyclic stress ratio to produce liquefaction in a treated sample over that of the untreated state, as a way of quantitatively expressing the amount of improvement, while also allowing a systematic comparison across different studies. The reported If values of some of the covered studies are summarized in Fig. 6. Overall, the cyclic improvement increased with higher treatment levels, although at different rates between experiments. The latter, in addition to some scatter at the same calcium carbonate levels, is due to the heterogeneity with regard to the geomaterial, treatment process, relative density, and testing apparatus used. A wider adoption of If could facilitate cross-study comparisons to reach a finer understanding and optimized conditions for MICP toward mitigating liquefaction.
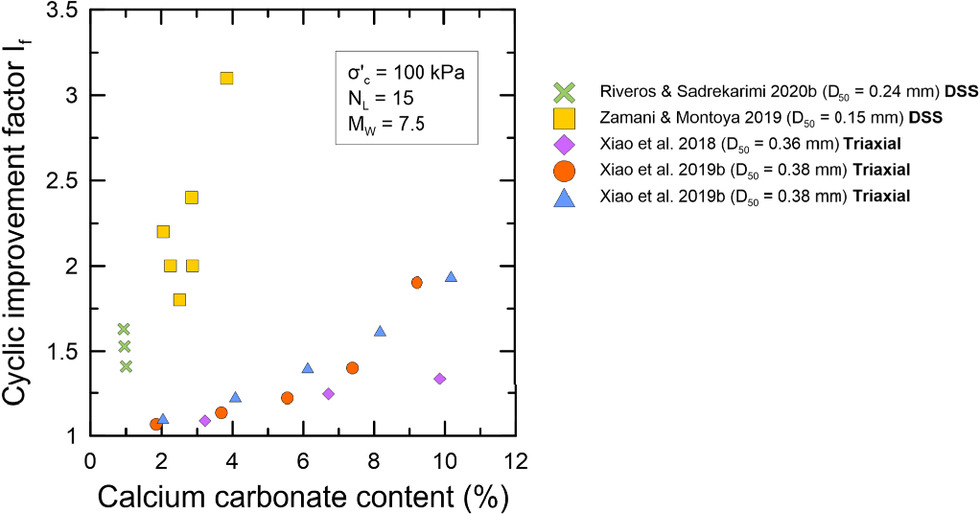
Alternatively, liquefaction mitigation is not exclusively addressed through mineralization, densification, or bonding but also through desaturation. Hence, microbially induced partial saturation was utilized in a series of DSS experiments, revealing that only a 4%–5% reduction in the degree of saturation could suffice to prevent liquefaction of sands with various silt contents (Mousavi and Ghayoomi 2021). Another study was able to bring such findings to site with field trials of microbially induced desaturation in low plasticity silts, in which, by extended monitoring, desaturation was seen to persist for more than 92 days after the intervention (Moug et al. 2022). By merging both approaches, microbially induced desaturation and precipitation (MIDP) has been recently gaining momentum; this method combines both desaturation due to biogenic gas production and carbonate precipitation to decrease the potential for earthquake-induced liquefaction of saturated granular soil (O’Donnell et al. 2019b). In a triaxial setup, Wang et al. (2021d) used MIDP to reduce the degree of saturation to 80%, while precipitating as little as 0.086% CaCO3. The liquefaction resistance then significantly improved, where NL increased by more than 25 times, while at a fixed 40 cycle threshold, the CRR doubled when compared with the untreated sample.
Modeling Aspects
Multiphysical Modeling
As a complement to experimental work, modeling may serve to investigate the specific principles of MICP in addition to serving as a predictive tool for designing treatment interventions. Most generally, the multiphysical phenomena governing MICP can be grouped into four principal mechanisms, namely, biological, chemical, hydrological, and mechanical. While the considered phenomena have remained largely unchanged since their early development (Fauriel and Laloui 2012; Hommel et al. 2015), recent studies have proposed a novel understanding of these core principles, often developing cross-scale formulations inspired by microscopic and macroscopic experimentations, such as for porosity, biochemical aspects, and field modeling.
Using a coupled biochemo-hydraulic model, Wang and Nackenhorst (2020) proposed effective and critical porosities as more accurate concepts to describe the evolution of materials with treatment compared with the commonly used porosity–permeability relationships. Other studies also highlighted the need to consider tortuosity, pore filling, and pore bridging by a variable surface area of CaCO3 crystals with other porosity models (Lin et al. 2020; Mehrabi and Atefi-Monfared 2021). From another perspective, the role of biological considerations was also recently addressed, specifically looking into the influence of cell concentration on the efficacy of MICP and its impact on the precipitation kinetics and the quality of the resulting crystals (Murugan et al. 2021). The study concluded that larger crystal sizes and a quicker transformation from vaterite to calcite could be achieved for slower and faster kinetics, respectively. Along the same lines, in another investigation that compared biochemical modeling with biogrouting experiments, an adjustment index was proposed to specifically consider the effective microbial biomass contributing to the reaction based on the measured discharge of calcium ions (Akiyama and Kawasaki 2019). From a more chemical perspective, Jain and Arnepalli (2020) investigated the impact of the pore fluid on the transport, adhesion, and deadhesion of ureolytic bacteria by combining column experiments with a model capable of quantifying interactions (attraction and repulsion) inspired by energy barrier problems. Nishimura and Matsubara (2021) proposed a bridging scheme in their model that coupled MICP (biochemical processes based on a reaction-diffusion system) with stress analysis, validating the creation of a new skeletal structure during treatment by filling pores and relaxing the stress concentration. Complementary multiphysics findings have been reported in studies focusing on EICP. For instance, in Kim et al. (2020), the use of a microfluidic experiment and its comparison with theoretical predictions revealed a possible overestimation of nucleation rates with supersaturation by the classical nucleation theory. Through EICP column tests, Hommel et al. (2020) proposed a model tailored for deep subsurface applications, accounting for nonisothermal reactive transport and both temperature-dependent enzymatically catalyzed ureolysis and urease inactivation.
Beyond pore and representative elementary volume (REV) scales, multiphysical models have been applied at meter-scale interventions. A multiphysical model was successfully used for the predictions and comparison with field observations in sealing a fractured sandstone formation using MICP (Cunningham et al. 2019). Additionally, relative to fracture clogging and 3D continuums, Minto et al. (2019) proposed modifications to different concepts, including attachment (velocity dependence or independence) and encapsulation. Specifically, the study also investigated the impact of an injection strategy, noting a higher homogeneity for a faster flow rate due to a further spread of reactants, while the use of a pressure control (instead of flow control) reduced the pressure heave near the surface and resulted in a better delivery of reactants. Zeng et al. (2021) analyzed an MICP field trial in fine soils using a 2D reactive transport model, which was capable of investigating potential sources of the lower reaction efficiency noted by the deployed monitoring strategies. For instance, the model confirmed the narrow spread of a treatment limited to 5% of the soil volume, while at the same time explaining the inhomogeneous distribution of bacteria and reactants outside of the treatment zone due to potential preferential flow paths and fracturing caused by the high pressures in impermeable clay layers. Finally, recent advances in coupled pore-scale electrokinetics and fluid flow in geomaterials to predict electroosmotic pressures from applied voltages could provide a strong modeling potential regarding the more recent EA-MICP or in understanding tortuosity and path complexity for MICP in general (Priya et al. 2021).
Constitutive Frameworks
The mechanical response of biocemented soils remains distinct from that of artificially cemented soils (using portland cement or lime), and depending on the initial, treatment and loading conditions, the fabric may result in a variety of responses within a soil-to-rock mechanics spectrum (Nafisi et al. 2020a; Wang et al. 2021a). Most elastoplastic constitutive models follow an identical skeletal framework to capture the mechanical response with treatment levels. Essentially, swelling in the yield surface of the untreated state is expressed following treatment, and destructuring formulations within the model are incorporated allowing the shrinkage of the treated yield surface back to its natural state, capturing the observed softening in the process, and eventually assuming no change to the critical state. It is in the definitions of the functions and parameters governing these two fundamental aspects, namely, the improvement and destructuring, that models diverge depending on their level of complexity and the chosen formulation scale (micro or macro). For instance, within their macroscopic model, Gai and Sánchez (2019) required only two MICP scaling constants, back-calculated from experimental results, to capture the improvement with regard to the increased cementation content and the damage rate on the mechanical contribution of precipitated calcite. In contrast, a microinspired model by Gajo et al. (2019) put forward a cross-scaled dependence of the stress–strain response on a cross-sectional area defined at the pore scale and a number of mechanically active bonds, defined using a simplified analytical geometry of spherical grains and cylindrical bonds. A closer examination of each model’s formulation also provides an insight into specific features. The use of the subloading concept, for example, allowed for the occurrence of irrecoverable strains while the stress state was still inside the main yield surface, resulting in a smoother transition between the elastic and the plastic behaviors and between compressive and dilative regimes (Gai and Sánchez 2019). Beyond a simple smoothening of responses, however, adopting subloading functions may provide a means to include fatigue-induced destructuring, caused by repetitive loading–unloading at a stress much lower than the effective peak of the main yield surface. Alternatively, creep effects can be accounted for, because the subloading concept is already used in modeling time-dependent soil phenomena (Liingaard et al. 2004). Gai and Sánchez (2019) also proposed a cementation level–dependent effective stress through an elastoplastic vector associated with CaCO3 dissolution or precipitation. Despite it being disregarded in the actual model, the suggestion that MICP could solely alter the effective stress state is a novel proposition, which warrants further investigation, because the concept goes against the nonintrusive characteristic generally attributed to a treatment. While the model of Gajo et al. (2019) is capable of capturing both naturally and artificially cemented geomaterials, destructuring or dissolution (mechanical or chemical loading) is a reducer of the number of active bonds. However, breaking the cementation within a structured soil is not equivalent to dissolving the precipitated CaCO3 in the lattice, passively coating grains, or filling pore spaces. Indeed, densification also plays a primary role in altering the mechanical response, even after breakage, in which the crushed fines may reduce particle contact stresses, redistribute the force chains in the soil skeleton, and dissipate energy through a cushioning effect (Xiao et al. 2020a). Few constitutive models, apart from that of Fauriel (2012), clearly differentiate the contribution of a given treatment into a coupled densification and bonding, while also considering both isotropic and deviatoric yield limits.
More generally, existing constitutive models from artificially cemented soils or for natural sands can also be adapted for biocementation. For instance, a critical state-based model with bounding surface plasticity (Boulanger and Ziotopoulou 2017) showed reasonable agreement when simulating monotonic and cyclic experimental results, while also suggesting that simple modifications of model parameters may not be sufficient to globally capture key features of a biotreated response (El Kortbawi et al. 2019). Another empirical approach proposed correlations for a nonlinear failure envelope, incorporating the impact of essential variables such as relative density, confinement, and soil characteristics on the friction angle (Nweke and Pestana 2018). Making use of the disturbed state concept (DSC), a constitutive model was developed for lignosulfonate-treated silty sand, which allows a direct adaptation for CaCO3 bonds within the same formulation (Athukorala et al. 2015). In addition to monotonic frameworks, Xiao et al. (2021c) proposed a cyclic constitutive model within the framework of nonlinear hyperelasticity and thermodynamics-based plasticity, hence bypassing the need for traditional modeling components such as a yield surface, plastic potential, and a loading–unloading criterion. Fundamentally, the work captured a high bond structure in the cyclic shear response of biocemented soils, even after the cohesion was fully degraded. Recently, unified constitutive models have more globally targeted all of the previously covered states simultaneously, namely, cemented and natural conditions, under both monotonic and cyclic loadings, capturing adequately qualitative and quantitative responses of experimental works under different loading conditions (Lu et al. 2022; Zhang et al. 2021c). A summary of the main discussed constitutive models, along with their formulation frameworks, parameters and other features, is given in Table 1.
Source | Model type and basis | Model framework | Scale domain | Tension considered | No. constitutive parameters | Originally developed for |
---|---|---|---|---|---|---|
Athukorala et al. (2015) | Mechanical elastoplastic DSC | Direct shear | Macro | No | 31 | Lignosulfonate |
Fauriel (2012) | Mechanical elastoplastic | Triaxial | Macro | No | 19 | MICP |
Gai and Sánchez (2019) | Mechanical elastoplastic | Triaxial | Macro | No | 8 | MICP |
Gajo et al. (2019) | Chemomechanical elastoplastic | Triaxial | Cross-scale (micro–macro) | Yes | 23 | Natural and MICP |
Koliji et al. (2008) | Mechanical elastoplastic | Oedometric | Macro | No | 5 | Structured soils |
Lu et al. (2022) | Mechanical elastoplastic | Triaxial monotonic and cyclic | Macro | Yes | 13 | Natural and MICP |
Xiao et al. (2021d) | Mechanical nonequilibrium thermodynamics | Triaxial cyclic | Macro | Yes | 14 | MICP |
Zhang et al. (2021c) | Mechanical nonequilibrium thermodynamics | Triaxial monotonic and cyclic | Macro | Yes | 13 | Natural and MICP |
Numerical Models
In addition to multiphysical and constitutive frameworks, numerical models enable a better understanding and prediction of biocemented soils and their complex behavior. While some recent studies have explored the use of machine learning and artificial intelligence algorithms (Maleki-kakelar et al. 2021; Wang and Yin 2021), most studies have relied on discrete-element methods (DEMs) in numerical simulations (Feng et al. 2017; Abbas et al. 2020). The latter are traditionally developed to elucidate particle-scale responses, in addition to serving as prediction tools at the macromechanical scale. Relative to bench-sized experiments of MICP-treated sands, Yang et al. (2019) used DEMs to simulate undrained triaxial conditions with flexible instead of rigid boundaries, using a cohesive bond strength to predict localization and shear band formation, in addition to capturing contact forces with varying cementation levels and axial strains. Along the same lines, in another investigation, stress–strain and failure behaviors in uniaxial loading were reproduced, but instead of assuming ideal spherical geometries, 3D morphology digitization of grains and bonds was employed (Wu et al. 2019), reporting a linear increase in strength with calcite content and a nonlinear decrease in strength with aggregate size. More fundamentally, Shen et al. (2019) made use of DEM simulations for damage characterization in structured sand by incorporating cross-scale macroscopic (stress and strain) and microscopic (contact force, relative position, and motion) quantities. Their results suggested a distinction between stress-based static damage and strain-dominated kinematic damage, categorized through directional damage indices such as anisotropic and isotropic, respectively. The damage process was also the focus of another investigation using a mesoscopic contact model with irregular crystal particles and an acoustic emission method for cemented sand, with a moment magnitude that reflected the damage process and fracture probability (Tang et al. 2019).
DEM simulations have also been used to investigate more specialized considerations relative to biocemented soils. For instance, the spatial and temporal variations in cementation on the mechanical response against controlled experiments were studied (Kashizadeh et al. 2021). Alternatively, in another study, the bending tensile response of a bonded soil was simulated (Rahman et al. 2021), while Duan et al. (2019) utilized a thermodynamic model to study the damage and crack propagation within biocemented sand subjected to different temperature drops or varying cooling durations. Through the use of photographic images, Zhang et al. (2021a) reconstructed real sand particles and CaCO3 bonds in their DEM model. The latter was capable of capturing the crucial role of crystal morphology between calcite and vaterite phases for the same content. In this study, large-scale applications could also be simulated, for instance, the penetration resistance of a biocemented crust, relative to desertification control. Additionally, within a large-scale mindset, in another investigation, a computationally effective DEM was used to validate the possibility of using MICP as an intervention technique to plug leakage pathways in the context of CO2 sequestration (Landa-Marbán et al. 2021). More recently, a DEM study could capture a near complete precipitation pattern, in which precipitates could be characterized as effective, partially effective, surface coating, and pore filling (Wu et al. 2023). This work allowed a bridging between the micro- and the macroscales with the proposition of different linear correlations between UCS and effective crystals for a range of variables such as cementation content and pretreatment porosity.
Upscaling Challenge
Scaling Perspectives
Despite significant progress made in laboratory characterization and predictive modeling, the reproducibility of such findings at the site scale remains crucial if the technique is to provide solutions for real-world problems. In many ways, the future of MICP is eventually determined by its large-scale feasibility. To tackle this upscaling challenge, one first needs to account for its various definitions and the considerations it encompasses.
If moving from the microanalyses to the REV scale constitutes an upscaling by itself, then, more generally, it is overall accepted that large-scale MICP experiments involve moving beyond the centimeter scale of REV relative to most soils to the meter scale (DeJong et al. 2010b). Indeed, the earliest attempts of scaling the technology utilized one-dimensional sand columns of conventional bench-sized diameters, reaching a meter in length (Van Paassen 2011; Martinez et al. 2013). Beyond the meter threshold, large-scale applications may include a wide range of spatial limits, from one-dimensional columns to two-dimensional radial flow problems relative to a cubic meter of soil (Van Paassen et al. 2009; Dejong et al. 2014), and last, to three-dimensional problems reaching tens or hundreds cubic meters of soil (Van Paassen et al. 2010; Esnault Filet et al. 2019).
From another perspective, upscaling experiments can also be differentiated between (1) controlled versus (2) open limit or free-field conditions. The former is usually a part of a reconstituted system, in which the geomaterials, treatment, and boundary conditions are chosen or closely controlled. In contrast, open limit conditions often refer to field trials or pilot projects, in which a treatment is adapted to a specific site in an attempt to address a geotechnical problem, with little to no control over the naturally existing soil and underground boundary conditions. Key findings of upscaling experiments reported in the literature in representative studies are summarized in Table 2. Generally, cross comparisons between different upscaling experiments remain complex. First, the number of conducted large-scale MICP attempts has been rather limited compared with the abundance of laboratory experiments due to the time and resources they require. In addition, each upscaling project is usually focused on the treatment of a different geomaterial using diverse solutions, concentrations, and treatment schemes, rendering each experiment effectively unique. Moreover, these experiments usually target or fit in the framework of a different envisaged MICP application.
Source | Soil volume or area considered | Treatment solutions, volumes, and concentrations BS, CS | Treatment technique | Monitoring techniques deployed | By-product consideration |
---|---|---|---|---|---|
Controlled boundary conditions | |||||
Van Paassen et al. (2009) | 2 × 1 m3 | BS: 0.1 m3 CS: 4 m3 (urea 0.5 M, calcium chloride 0.5 M) | Injection | UCS: 0–9 MPa Manual penetrometer: 5 MPa | No |
Gomez et al. (2017) | 2 × 1.2 m3 | BS: 0.6 m3 CS: 1.3 m3 (urea 0.35 M, calcium chloride 0.25 M) | Injection | Biological sampling Vs: 145–950 m/s Cone penetration: 5–25 MPa | No |
Van Paassen et al. (2010) | 100 m3 | BS: 5 m3 CS: 96 m3 (urea 1 M, calcium chloride 1 M) | Injection | UCS: 0.7–12 MPa Eur: 0.1–13 GPa Vs: 188–395 m/s | Yes 30 m3 fresh water rinse |
Esnault-Filet et al. (2012) | 100 m3 | BS: 15 m3 CS: — | Injection | Sampling boreholes UCS: 50–500 kPa Triaxial: φ′ = 36°, c′ = 54 kPa Permeability: same order magnitude 10−4 m/s | No |
Esnault Filet et al. (2019) | 4 × 45–70 m3 | BS: — CS: — | Injection | Penetrometer: 3–50 MPa Vs: 300–1,300 m/s Triaxial: φ′ = 53°, c′ = 104 kPa Jet erosion test: τc = 0.04–1.5 kPa Hole erosion test: τc = 0.23–2 kPa | Yes Multiple flushing days |
Open-field boundary conditions | |||||
Van Paassen (2011) | 2 × 1,000 m3 Borehole stabilization | BS: 200 m3 CS: 600 m3 | Injection | Chemical and Vs monitoring CPT, SPT Electric resistivity: 2–15 Ω m | Yes Pumping and wastewater treatment |
Esnault-Filet et al. (2016) | 100 m3 Infrastructure rehabilitation | — | Injection | — | No |
Gomez et al. (2015) | 35 m2 Erosion protection | BS: 5.7 m3 CS: 17 m3 (3 different concentrations fixed 2:1 ratio urea-calcium chloride) | Surface spraying | Dual mass cone penetration: 2–4 blows/cm Discrete sampling | No |
Ghasemi and Montoya (2020) | 8 m2 Erosion protection | BS: 1.2 m3 CS: 4.5 m3 (urea 0.3 M, calcium chloride 0.1 M) | Surface spraying | Dual mass cone penetration: 3.5 cm/blow Percolation test: preserved surface permeability k = 4.2 × 10−5 m/s | No |
Terzis et al. (2020b) | 51 m2 Slope stabilization | BS: 2 m3 CS: 2 m3 (urea 0.05 M, calcium chloride 0.04 M) | Injection | Discrete sampling Aerial optical surveillance displacement monitoring | No |
Note: BS = bacterial dolution; CS = cementation solution; and — = no data available.
Notable Attempts and Findings
Among the controlled boundary experiments, Van Paassen et al. (2009) adapted a 1 m3 multibox container setup with drainage filters on the side to mimic a spherical injection point and used it to treat two sands applying 3,500 and 4,000 L of reagent solutions, respectively. CaCO3 ranging from 100–250 kg/m3 precipitated in the sand box, yielding manual cone penetration resistances higher than 5 MPa near the surface and UCS values reaching 9 MPa. Gomez et al. (2017, 2018) built twin tanks each with a 1.2 m3 capacity but with finer boundary control through imposed flow regulation and the addition of aqueous sampling ports. For bioaugmentation treatment, consisting of six pore volumes of cementation solution, the maximum calcite content reached 5% in certain locations, corresponding to a Vs of 950 m/s and a penetration tip resistance reaching 25 MPa. Notable heterogeneity was also observed, with the lowest values falling within the untreated envelope at 130 m/s and 5 MPa. Even larger concrete tanks were constructed with a 100 m3 capacity, for instance, by Van Paassen et al. (2010). The treatment scale was reflected in the large volumes mobilized, namely, 5 m3 of the bacterial suspension and 96 m3 of the urea-calcium chloride reagent solution. At the conclusion of biogrouting, the Vs values varied between 188 and 395 m/s depending on the location. Only highly cemented samples could be cored, with contents ranging between 12% and 27%; the UCS values ranged from 0.7 to 12 MPa, and Young’s modulus values ranged between 0.1 and 13 GPa. A similar trial was conducted by Esnault-Filet et al. (2012) in treating 100 m3 of the same sand, from which 110 cored samples yielded a conservative UCS average value of 200 kPa, considering dispersion in the results. In addition, triaxial tests revealed that the treatment had almost no impact on the angle of internal shear resistance between natural (33°) and treated cases (32° and 36°), but that cohesion could reach values of approximately 50 kPa following the treatment, while hydraulic conductivity remained in the range of 10−4 m/s. In another concrete basin with 70 m3 capacity, Esnault Filet et al. (2019) concluded four different tests with varying geomaterials, targeting homogeneous and layered soils with interfaces, often subject to internal erosion. Disclosed results reveal successful improvements, with Vs and penetrometer resistance values of 300–1,370 m/s and 3–50 MPa, respectively, and a net increase in the erosion resistance (τc = 40–2,000 Pa). The study also captured the minimal impact of MICP on the living soil compared with other soil improvement techniques by comparing the average oxygen consumption per day in the soil after treatment (18–24 mg O2 consumed/day compared with the 20 mg/day of the reference state).
From another perspective, relative to open limit conditions, several projects were notably reported in more than five countries. More precisely, in the Netherlands, following a successful test on 3 m3 of gravel in the laboratory, two field tests relied on biogrouting to treat 1,000 m3 of soil each at depths between 3 and 20 m as part of a gas pipeline installation project to mitigate potential borehole collapse (Van Paassen 2011), monitored by means of chemical analyses, Vs measurements, and CPT and SPT tests. In France, a sandy-silt material was treated beneath the abutment of a motorway interchange bridge in a crowded urban area with limited access (Esnault-Filet et al. 2016), by drilling 23 horizontal wells in a mesh covering a total volume equal to 100 m3. At a mine site in Canada, a superficial application of MICP aimed to increase erosion resistance, surficial stability, and dust abatement and to potentially enable revegetation (Gomez et al. 2015) with the spraying of 22.7 m3 solutions over a total surface of 35 m2. The results captured the formation of a 0.6–2 cm thick crust with an increase in dual-mass dynamic cone penetrometer blow counts (2–3 blows per cm) for the same fixed energy. Additionally, addressing the issue of surface erodibility, an MICP treatment based on nozzle spraying of 5.7 m3 of solutions was used on an 8 m2 coastal sandy slope in the United States (Ghasemi and Montoya 2022), resulting in precipitation reaching 18–20 cm below the surface and a reduction in the penetration index from an average of 9–3.5 cm/blow. Similar findings were made in another work, in which MICP could significantly improve the erosion pattern of slopes for rainfall intensities as high as 175 mm/h (Xiao et al. 2022d). Additionally, in the United States, following laboratory optimization studies, an MICP recipe was upscaled under nonideal conditions to verify its performance in preventing soil erosion compared with competing technologies (Hodges and Lingwall 2020). In Switzerland, the novel MICP technique based on ex situ hydrolysis was deployed by injecting 4 m3 of solutions to mitigate landslide risk by inducing biomineralization of calcite binders within the targeted slip zone, coupled with more typical drainage trenches (Terzis et al. 2020b), in which, through optical drone surveillance, a slower movement of the slope was recorded in the MICP-treated zone compared with the surrounding untreated areas.
Specific Upscaling Considerations
Considering the aforementioned, aside from the change in the order of magnitude of scales (from mm3 to m3), upscaling attempts also constitute a change in paradigm in contrast to bench studies. In addition to the challenge of preparing, transporting, and deploying large volume of solutions, new considerations arise that are usually ignored or are nonexistent at the laboratory scale, such as homogeneity, quality control, by-product elimination, cost efficiency, and treatment permanence.
At a large scale, ensuring a homogeneous distribution of precipitated calcium carbonate is more complicated. Indeed, a 3D flow is established around the injection–extraction wells, with anisotropic permeability that also depends on the pumping rates, reactive transport, and changing tortuosity. Additionally, the erosion that can take place between the treatment wells and the soil may contribute to some heterogeneity, which leads to the development of more advanced delivery systems (Do et al. 2020). Localization effects with over- or undertreated zones can result in poorly performing sections within the targeted geologic layer, impacting the overall ultimate or serviceability performance.
As such, quality control is a central aspect of any upscaling attempt to monitor the proper progress of underground treatment. Multiple destructive and nondestructive monitoring techniques, such as pH, electric conductivity (EC), bacterial count, urease activity, and precipitated CaCO3 measurements, can be employed to validate the prevalence of adequate biochemohydro conditions needed to ensure high precipitation efficiency (Gomez et al. 2019). Alternatively, monitoring techniques can target modification in the mechanical response through direct or indirect assessment, for instance, by means of geophysical, in situ, or site mockup tests. More precisely, geophysical techniques include shear-wave velocity (Van Paassen et al. 2010; Gomez et al. 2017; Esnault Filet et al. 2019) and electrical resistivity (Van Paassen 2011). On the other hand, common in situ strength assessments generally rely on penetration techniques using penetrometers of different sizes and instrumentalization (Esnault Filet et al. 2019; Ghasemi and Montoya 2020; Gomez et al. 2015, 2018). Using mockup models of geomaterials (meter-scaled samples), optimal site treatment conditions could be identified using methods such as the California bearing ratio and dynamic deformation modulus tests (Xiao et al. 2022c; Xu et al. 2023).
In consideration of the quantities of involved reactants in large-scale experiments, significant amounts of by-products can be expected. Most notably, ammonium is the main undesired side product of the hydrolysis reaction, which, without proper remediation, results in contamination of the soil and underground water. While traditional flushing with excess fresh water (>5–10 pore volumes) has been the norm in the laboratory to remove excess salts and undesired by-products, relying on the same strategy at the scale of real geotechnical works is not always feasible or sustainable. This is due to the residual presence of ammonium or the large amounts of fresh water that would be required to dilute its concentration to acceptable limits. One should consider herein that there exists regulations regarding the water quality criteria thresholds for ammonia (chronic total ammonium nitrogen 1.9 mg/L) (USEPA 2013). Such an attempt is reported in the literature in a large-scale MICP application, which required continuous groundwater extraction until the injected ammonium was fully recovered, while the pumped fresh water volume was sent to a wastewater treatment plant (Van Paassen 2011). More recent approaches propose alternative treatment strategies that allow capturing of ammonium and recycling it in the form of soil fertilizer, thereby turning an undesired by-product into a valorized resource (Terzis and Laloui 2021).
An additional challenge relative to large-scale applications is the issue of cost. The low-pressure injections, low-viscosity solutions, and rapidity of the process, convey a competitive edge to MICP especially compared with typical cement or resin grouting approaches. Even the choice of reactants, equipment, and treatment design (scheme, number of injection days, concentrations, etc.) must remain cost-effective and cost-competitive. As such, upscaling attempts strive to maintain high efficiency reactions despite the use of lower-grade industrial chemicals instead of synthesized ones (e.g., deicing salts as a source of calcium chloride that are available in large quantities but often come with minimal quality control and large fluctuation in purity); this approach is similar with regard to the choice of commercial and contractor-friendly equipment (Do et al. 2020; Esnault-Filet et al. 2012; Esnault Filet et al. 2019; Ghasemi and Montoya 2020).
Finally, in upscaled field trials or pilot projects, retention of the enhanced features conveyed by the intervention is fundamental. In contrast to lab-prepared specimens that are immediately tested after treatment, as part of a targeted mechanical, chemical, or other types of characterization, site applications need to exhibit a treatment permeance. Resilience in soil improvement is therefore necessary against environmental conditions, such as changes in pH, temperature, and infiltration of acid rain, over the life span of the civil system or until a maintenance intervention is foreseen (DeJong et al. 2013; Haouzi and Courcelles 2018; Dejong and Kavazanjian 2019).
Toward Mainstream Applications
Mainstream applications of biocementation could benefit from standardization in the design process. To date, there have been limited design tools or decision aids that can serve as a solid starting point in assisting engineers working with biomineralization for soil reinforcement. Moreover, existing standards and codes relative to traditional soil grouting may not be adequate in structure (verification steps) or metrics (factors of safety) to accommodate the specificities of biocementation, whose governing variables are no longer the viscosity, water-cement ratio, or curing time, compared with traditional injection slurries.
As a final note, a decade after some 24 biogeochemical applications of MICP were envisaged and ranked (DeJong et al. 2013), a refocus of the research and applied efforts toward the main applications is currently needed to assist the adoption of biocementation as a mainstream soil improvement strategy. Drawing a parallel from similar novel concepts in the field, such as artificial ground freezing (Alzoubi et al. 2020), reveals that the strong potential of MICP could lie in unconventional rather than in mainstream applications. Conditions in which biogrouting could inherently hold a competitive edge, compared with traditional alternatives, typically include scenarios with permeable granular materials and low required cementation levels. For example, liquefaction mitigation requires calcite contents even below 1%, which is adequate to endow geomaterials with improved cohesion in the order of 10–30 kPa.
Conclusions
This work puts the focus on biocementation as one of the most promising biomediated solutions for soil improvement. Following decades of research, a holistic evaluation of the core and milestone findings of the technique is presented. First, the fundamentals of the biochemical processes relative to MICP are covered, ranging from engineering, microbiology, and geochemistry and from the microscale to the macroscale. This is more elaborately followed by a structured and in-depth comprehension of the main geomechanical considerations. Precisely, the mechanics, modeling, and upscaling efforts, currently governing the application and performance of biocementation, are addressed in the respective sections. A synthesis of recently reported trends in mechanical response is further presented and discussed. Overall, the scattered mechanical response is believed to highlight the need to categorize geomaterials based on granulometry or relative density to ultimately interpret the often-considered treatment differences or provide adapted treatment recipes. The paper stresses the need to move beyond the use of CaCO3 content as the sole indicator of the improved material properties and suggests incorporating quantities that reflect the properties of the pretreatment condition of geomaterials because they seem to govern the boundaries of the expected improvement. For results obtained within comparable conditions, a steady convergence toward similar trends is observed, which can eventually result in a better standardization in behavioral predictions and modeling efforts. The latter are found to benefit from improved complex mathematical formulations and better microstructural understanding to ultimately incorporate modeling parameters that reflect the physical mechanisms involved at the microscale. The contribution of upscaling efforts, while by far the most diversified aspect studied in this paper, is found to be significant toward capturing, reporting, and understanding the limitations and obstacles of the technique, with a specific focus on the problem of residual ammonium. Overall, the synthesis of the presented recent progress aims to identify and prepare the next pivotal improvements as biocementation reaches new maturity levels toward industry applications.
Data Availability Statement
All data, models, and codes generated or used during the study appear in the published article. The SEM images and additional data for possible discussions and comparisons are available from the corresponding author upon reasonable request.
Acknowledgments
The authors wish to thank the European Research Council (ERC) for the financial support under the European Union’s Horizon 2020 research and innovation program (Grant Agreement No.: 788587).
References
Abbas, M., D. Kunhappan, A. Dadda, C. Geindreau, F. Emeriault, A. Esnault-Filet, and A. Garandet. 2020. “Discrete element modelling for biocemented sand: Effect of calcite distribution at the microscopic scale.” E3S Web Conf. 195: 05005. https://doi.org/10.1051/e3sconf/202019505005.
Ahenkorah, I., M. M. Rahman, M. R. Karim, and P. R. Teasdale. 2020. “A comparison of mechanical responses for microbial-and enzyme-induced cemented sand.” Geotech. Lett. 10 (4): 559–567. https://doi.org/10.1680/jgele.20.00061.
Akiyama, M., and S. Kawasaki. 2019. “Biogeochemical simulation of microbially induced calcite precipitation with Pararhodobacter sp. strain SO1.” Acta Geotech. 14 (3): 685–696. https://doi.org/10.1007/s11440-019-00784-z.
Almajed, A., M. A. Lateef, A. A. B. Moghal, and K. Lemboyer. 2021. “State-of-the-art review of the applicability and challenges of microbial-induced calcite precipitation (MICP) and enzyme-induced calcite precipitation (EICP) techniques for geotechnical and geoenvironmental applications.” Crystals 11 (370): 1–22.
Alzoubi, M. A., M. Xu, F. P. Hassani, S. Poncet, and A. P. Sasmito. 2020. “Artificial ground freezing: A review of thermal and hydraulic aspects.” Tunnelling Underground Space Technol. 104: 103534. https://doi.org/10.1016/j.tust.2020.103534.
Arboleda-Monsalve, L. G., D. G. Zapata-Medina, and D. I. Galeano-Parra. 2019. “Compressibility of biocemented loose sands under constant rate of strain, loading, and pseudo K0-triaxial conditions.” Soils Found. 59 (5): 1440–1455. https://doi.org/10.1016/j.sandf.2019.06.008.
Athukorala, R., B. Indraratna, and J. S. Vinod. 2015. “Disturbed state concept-based constitutive model for lignosulfonate-treated silty sand.” Int. J. Geomech. 15 (6): 04015002. https://doi.org/10.1061/(asce)gm.1943-5622.0000473.
Boulanger, R. W., and K. Ziotopoulou. 2017. PM4Sand (version 3.1): A sand plasticity model for earthquake engineering applications. Davis, CA: Dept. of Civil and Environmental Engineering, College of Engineering, Univ. of California.
Burdalski, R. J., B. G. O. Ribeiro, M. G. Gomez, and D. Gorman-Lewis. 2022. “Mineralogy, morphology, and reaction kinetics of ureolytic bio-cementation in the presence of seawater ions and varying soil materials.” Sci. Rep. 12 (1): 1–24. https://doi.org/10.1038/s41598-022-21268-3.
Cardoso, R., E. Arbabzadeh, J. T. de Lima, I. Flores-Colen, M. F. C. Pereira, M. Costa e Silva, S. O. D. Duarte, and G. A. Monteiro. 2021. “The influence of stone joints width and roughness on the efficiency of biocementation sealing.” Constr. Build. Mater. 283: 122743. https://doi.org/10.1016/j.conbuildmat.2021.122743.
Chen, M., S. Gowthaman, K. Nakashima, and S. Kawasaki. 2021. “Evaluating mechanical strength of peat soil treated by fiber incorporated bio-cementation.” Int. J. GEOMATE 20 (78): 121–127. https://doi.org/10.21660/2021.78.Gx162.
Cheng, L., R. Cord-Ruwisch, and M. A. Shahin. 2013. “Cementation of sand soil by microbially induced calcite precipitation at various degrees of saturation.” Can. Geotech. J. 50 (1): 81–90. https://doi.org/10.1139/cgj-2012-0023.
Cheng, L., T. Kobayashi, and M. A. Shahin. 2020. “Microbially induced calcite precipitation for production of ‘bio-bricks’ treated at partial saturation condition.” Constr. Build. Mater. 231: 117095. https://doi.org/10.1016/j.conbuildmat.2019.117095.
Choi, S. G., I. Chang, M. Lee, J. H. Lee, J. T. Han, and T. H. Kwon. 2020. “Review on geotechnical engineering properties of sands treated by microbially induced calcium carbonate precipitation (MICP) and biopolymers.” Constr. Build. Mater. 246: 118415. https://doi.org/10.1016/j.conbuildmat.2020.118415.
Choi, S.-G., T. Hoang, E. J. Alleman, and J. Chu. 2019. “Splitting tensile strength of fiber-reinforced and biocemented sand.” J. Mater. Civ. Eng. 31 (9): 06019007. https://doi.org/10.1061/(asce)mt.1943-5533.0002841.
Choi, S. G., K. Wang, and J. Chu. 2016. “Properties of biocemented, fiber reinforced sand.” Constr. Build. Mater. 120: 623–629. https://doi.org/10.1016/j.conbuildmat.2016.05.124.
Clarà Saracho, A., S. K. Haigh, and M. Ehsan Jorat. 2020a. “Flume study on the effects of microbial induced calcium carbonate precipitation (MICP) on the erosional behaviour of fine sand.” Géotechnique 71: 12. https://doi.org/10.1680/jgeot.19.p.350.
Clarà Saracho, A., S. K. Haigh, T. Hata, K. Soga, S. Farsang, S. A. T. Redfern, and E. Marek. 2020b. “Characterisation of CaCO3 phases during strain-specific ureolytic precipitation.” Sci. Rep. 10 (1): 1–12. https://doi.org/10.1038/s41598-020-66831-y.
Clarà Saracho, A., L. Lucherini, M. Hirsch, H. M. Peter, D. Terzis, E. Amstad, and L. Laloui. 2021. “Controlling the calcium carbonate microstructure of engineered living building materials.” J. Mater. Chem. A 9 (43): 24438–24451. https://doi.org/10.1039/d1ta03990c.
Consoli, N. C., R. C. Cruz, M. F. Floss, and L. Festugato. 2010. “Parameters controlling tensile and compressive strength of artificially cemented sand.” J. Geotech. Geoenviron. Eng. 136 (5): 759–763. https://doi.org/10.1061/(asce)gt.1943-5606.0000278.
Cunningham, A. B., H. Class, A. Ebigbo, R. Gerlach, A. J. Phillips, and J. Hommel. 2019. “Field-scale modeling of microbially induced calcite precipitation.” Comput. Geosci. 23 (2): 399–414. https://doi.org/10.1007/s10596-018-9797-6.
Dadda, A., C. Geindreau, F. Emeriault, A. Esnault Filet, and A. Garandet. 2019a. “Influence of the microstructural properties of biocemented sand on its mechanical behavior.” Int. J. Numer. Anal. Methods Geomech. 43 (2): 568–577. https://doi.org/10.1002/nag.2878.
Dadda, A., C. Geindreau, F. Emeriault, S. Rolland du Roscoat, A. Esnault Filet, and A. Garandet. 2019b. “Characterization of contact properties in biocemented sand using 3D X-ray micro-tomography.” Acta Geotech. 14 (3): 597–613. https://doi.org/10.1007/s11440-018-0744-4.
Darby, K. M., G. L. Hernandez, J. T. DeJong, R. W. Boulanger, M. G. Gomez, and D. W. Wilson. 2019. “Centrifuge model testing of liquefaction mitigation via microbially induced calcite precipitation.” J. Geotech. Geoenviron. Eng. 145 (10): 04019084. https://doi.org/10.1061/(asce)gt.1943-5606.0002122.
Das, B. M., S. C. Yen, and R. N. Dass. 1995. “Brazilian tensile strength test of lightly cemented sand.” Can. Geotech. J. 32 (1): 166–171. https://doi.org/10.1139/t95-013.
Dejong, J. T., and E. Kavazanjian. 2019. “Bio-mediated and bio-inspired geotechnics.” In Geotechnical fundamentals for addressing new world challenges, edited by N. Lu and J. Mitchell, 193–207. Cham, Switzerland: Springer.
Dejong, J. T., B. C. Martinez, T. R. Ginn, C. Hunt, D. Major, and B. Tanyu. 2014. “Development of a scaled repeated five-spot treatment model for examining microbial induced calcite precipitation feasibility in field applications.” Geotech. Test. J. 37 (3): 424–435. https://doi.org/10.1520/GTJ20130089.
DeJong, J. T., B. M. Mortensen, B. C. Martinez, and D. C. Nelson. 2010a. “Bio-mediated soil improvement.” Ecol. Eng. 36 (2): 197–210. https://doi.org/10.1016/j.ecoleng.2008.12.029.
DeJong, J. T., K. Soga, S. A. Banwart, W. R. Whalley, T. R. Ginn, D. C. Nelson, B. M. Mortensen, B. C. Martinez, and T. Barkouki. 2010b. “Soil engineering in vivo: Harnessing natural biogeochemical systems for engineering solutions.” J. R. Soc. Interface 8 (54): 1–15.
DeJong, J. T., et al. 2013. “Biogeochemical processes and geotechnical applications: Progress, opportunities and challenges.” Géotechnique 63 (4): 287–301. https://doi.org/10.1680/geot.SIP13.P.017.
Dhami, N. K., A. Mukherjee, and M. S. Reddy. 2016. “Micrographical, minerological and nano-mechanical characterisation of microbial carbonates from urease and carbonic anhydrase producing bacteria.” Ecol. Eng. 94: 443–454. https://doi.org/10.1016/j.ecoleng.2016.06.013.
Dikshit, R., A. Dey, N. Gupta, S. C. Varma, I. Venugopal, K. Viswanathan, and A. Kumar. 2021. “Space bricks: From LSS to machinable structures via MICP.” Ceram. Int. 47 (10): 14892–14898. https://doi.org/10.1016/j.ceramint.2020.07.309.
Ding, J., Z. Wang, N. Zhang, P. Jiang, M. Peng, Y. Jin, and Q. Li. 2019. “Experimental study on thermal conductivity of sand solidified by microbially induced calcium carbonate precipitation.” IOP Conf. Ser.: Earth Environ. Sci. 340 (5): 052069. https://doi.org/10.1088/1755-1315/304/5/052069.
Do, J., B. M. Montoya, and M. A. Gabr. 2019. “Debonding of microbially induced carbonate precipitation-stabilized sand by shearing and erosion.” Geomech. Eng. 17 (5): 429–438. https://doi.org/10.12989/gae.2019.17.5.429.
Do, J., B. M. Montoya, and M. A. Gabr. 2020. “Scour mitigation and erodibility improvement using microbially induced carbonate precipitation.” Geotech. Test. J. 44 (5): 20190478. https://doi.org/10.1520/GTJ20190478.
Duan, Y., C. Kim, and G. Xu. 2019. “Temperature drop model based on discrete element method for simulating damage of bio-cemented sand by cold wave.” J. Shanghai Jiaotong Univ. (Sci.) 24 (6): 789–798. https://doi.org/10.1007/s12204-019-2123-1.
El Kortbawi, M., K. Ziotopoulou, M. G. Gomez, and M. Lee. 2019. “Validation of a bounding surface plasticity model against the experimental response of (bio-) cemented sands.” In Geo-Congress 2019: Earthquake Engineering and Soil Dynamics, Geotechnical Special Publication 308, edited by C. L. Meehan, S. Kumar, M. A. Pando, and J. T. Coe, 197–207. Reston, VA: ASCE.
Elmaloglou, A., D. Terzis, P. De Anna, and L. Laloui. 2022. “Microfluidic study in a meter-long reactive path reveals how the medium’s structural heterogeneity shapes MICP-induced biocementation.” Sci. Rep. 12 (1): 1–16. https://doi.org/10.1038/s41598-022-24124-6.
Esnault-Filet, A., J.-P. Gadret, L. Memphis, and S. Borel. 2012. “Biocalcis and its applications for the consolidation of sands.” In Grouting and Deep Mixing, Geotechnical Special Publication 228, edited by L. F. Johnsen, D. A. Bruce, and M. J. Byle, 1767–1780. Reston, VA: ASCE.
Esnault-Filet, A., I. Gutjahr, J. Mosser, L. Sapin, and K. Ibrahim. 2016. “A novel grouting process for the reinforcement of low permeability soils with the use of biocementation by biocalcis.” In Proc., Southeast Asian Geotechnical Conf. & 2nd AGSSEA Conf. Kuala Lumpur, Malaysia: Association of Geotechnical Societies in Southeast Asia (AGSSEA).
Esnault Filet, A., et al. 2019. “BOREAL, bio-reinforcement of embankments by biocalcification.” In Proc., Digues Maritimes et Fluviales de Protection contre les Inondations, 1–10. France: IRSTEA.
Fang, X., Y. Yang, Z. Chen, H. Liu, Y. Xiao, and C. Shen. 2020. “Influence of fiber content and length on engineering properties of MICP-treated coral sand.” Geomicrobiol. J. 37 (6): 582–594. https://doi.org/10.1080/01490451.2020.1743392.
Fatehi, H., D. E. L. Ong, J. Yu, and I. Chang. 2021. “Biopolymers as green binders for soil improvement in geotechnical applications: A review.” Geosciences (Switzerland) 11 (7): 1–39. https://doi.org/10.3390/geosciences11070291.
Fauriel, S. 2012. Multiphysical modelling of soils with a focus on microbially induced calcite precipitation. Lausanne, Switzerland: Ecole Polytechnique Fédérale de Lausanne (EPFL).
Fauriel, S., and L. Laloui. 2012. “A bio-chemo-hydro-mechanical model for microbially induced calcite precipitation in soils.” Comput. Geotech. 46: 104–120. https://doi.org/10.1016/j.compgeo.2012.05.017.
Feng, K., and B. M. Montoya. 2014. “Behavior of bio-mediated soil in k0 loading.” In New Frontiers in Geotechnical Engineering, Geotechnical Special Publication 243, edited by G. Zhang and Z. Liu, 1–10. Reston, VA: ASCE.
Feng, K., and B. M. Montoya. 2016. “Influence of confinement and cementation level on the behavior of microbial-induced calcite precipitated sands under monotonic drained loading.” J. Geotech. Geoenviron. Eng. 142 (1): 04015057. https://doi.org/10.1061/(asce)gt.1943-5606.0001379.
Feng, K., B. M. Montoya, and T. M. Evans. 2017. “Discrete element method simulations of bio-cemented sands.” Comput. Geotech. 85: 139–150. https://doi.org/10.1016/j.compgeo.2016.12.028.
Gai, X., and M. Sánchez. 2019. “An elastoplastic mechanical constitutive model for microbially mediated cemented soils.” Acta Geotech. 14: 709–726. https://doi.org/10.1007/s11440-018-0721-y.
Gajo, A., F. Cecinato, and T. Hueckel. 2019. “Chemo-mechanical modeling of artificially and naturally bonded soils.” Geomech. Energy Environ. 18: 13–29. https://doi.org/10.1016/j.gete.2018.11.005.
Gao, K., H. Lin, M. T. Suleiman, P. Bick, T. Babuska, X. Li, J. Helm, D. G. Brown, and N. Zouari. 2023. “Shear and tensile strength measurements of CaCO3 cemented bonds between glass beads treated by microbially induced carbonate precipitation.” J. Geotech. Geoenviron. Eng. 149 (1): 1–14. https://doi.org/10.1061/(asce)gt.1943-5606.0002927.
Gao, Y. F., L. Hang, J. He, F. Zhang, and L. Van Paassen. 2021. “Pullout behavior of geosynthetic reinforcement in biocemented soils.” Geotext. Geomembr. 49 (3): 646–656. https://doi.org/10.1016/j.geotexmem.2020.10.028.
Ghasemi, P., and B. M. Montoya. 2020. “Field application of the microbially induced calcium carbonate precipitation on a coastal sandy slope.” In Geo-Congress 2020: Biogeotechnics, Geotechnical Special Publication 320, edited by E. Kavazanjian, J. P. Hambleton, R. Makhnenko, and A. S. Budge, 141–149. Reston, VA: ASCE.
Ghasemi, P., and B. M. Montoya. 2022. “Field implementation of microbially induced calcium carbonate precipitation for surface erosion reduction of a coastal plain sandy slope.” J. Geotech. Geoenviron. Eng. 148 (9): 04022071. https://doi.org/10.1061/(asce)gt.1943-5606.0002836.
Gleaton, J., Z. Lai, R. Xiao, Q. Chen, and Y. Zheng. 2019. “Microalga-induced biocementation of Martian regolith simulant: Effects of biogrouting methods and calcium sources.” Constr. Build. Mater. 229: 116885. https://doi.org/10.1016/j.conbuildmat.2019.116885.
Gomez, M. G., C. M. Anderson, C. M. R. Graddy, J. T. DeJong, D. C. Nelson, and T. R. Ginn. 2017. “Large-scale comparison of bioaugmentation and biostimulation approaches for biocementation of sands.” J. Geotech. Geoenviron. Eng. 143 (5): 04016124. https://doi.org/10.1061/(ASCE)GT.1943-5606.0001640.
Gomez, M. G., J. T. Dejong, and C. M. Anderson. 2018. “Effect of bio-cementation on geophysical and cone penetration measurements in sands.” Can. Geotech. J. 55 (11): 1632–1646. https://doi.org/10.1139/cgj-2017-0253.
Gomez, M. G., C. M. R. Graddy, J. T. DeJong, and D. C. Nelson. 2019. “Biogeochemical changes during bio-cementation mediated by stimulated and augmented ureolytic microorganisms.” Sci. Rep. 9 (1): 1–15. https://doi.org/10.1038/s41598-018-37186-2.
Gomez, M. G., B. C. Martinez, J. T. DeJong, C. E. Hunt, L. A. DeVlaming, D. W. Major, and S. M. Dworatzek. 2015. “Field-scale bio-cementation tests to improve sands.” Proc. Inst. Civ. Eng. Ground Improv. 168 (3): 206–216. https://doi.org/10.1680/grim.13.00052.
Gowthaman, S., M. Chen, K. Nakashima, and S. Kawasaki. 2021. “Effect of scallop powder addition on MICP treatment of amorphous peat.” Front. Environ. Sci. 9: 1–13. https://doi.org/10.3389/fenvs.2021.690376.
Graddy, C. M. R., M. G. Gomez, J. T. Dejong, and D. C. Nelson. 2021. “Native bacterial community convergence in augmented and stimulated ureolytic MICP biocementation.” Environ. Sci. Technol. 55: 15. https://doi.org/10.1021/acs.est.1c01520.
Graddy, C. M. R., M. G. Gomez, L. M. Kline, S. R. Morrill, J. T. Dejong, and D. C. Nelson. 2018. “Diversity of sporosarcina-like bacterial strains obtained from meter-scale augmented and stimulated biocementation experiments.” Environ. Sci. Technol. 52 (7): 3997–4005. https://doi.org/10.1021/acs.est.7b04271.
Ham, S.-M., A. Martinez, G. Han, and T.-H. Kwon. 2022. “Grain-scale tensile and shear strengths of glass beads cemented by MICP.” J. Geotech. Geoenviron. Eng. 148 (9): 1–14. https://doi.org/10.1061/(asce)gt.1943-5606.0002863.
Haouzi, F.-Z., and B. Courcelles. 2018. “Major applications of MICP sand treatment at multi-scale levels: A review.” In Proc., GeoEdmonton. Edmonton, Canada: The Geotechnical Society of Edmonton.
Haouzi, F.-Z., A. Esnault-Filet, and B. Courcelles. 2018. “Performance studies of microbial induced calcite precipitation to prevent the erosion of internally unstable granular soils.” In Civil infrastructures confronting severe weathers and climate changes: From failure to sustainability, edited by D. Chen, S. Kim, and A. Tapase, 37–49. Cham, Switzerland: Springer.
Haouzi, F. Z., A. Esnault-Filet, and B. Courcelles. 2020. “Optimisation of microbially induced calcite precipitation protocol against erosion.” Environ. Geotech. 40: 1–12. https://doi.org/10.1680/jenge.19.00083.
Harkes, M. P., L. A. van Paassen, J. L. Booster, V. S. Whiffin, and M. C. M. van Loosdrecht. 2010. “Fixation and distribution of bacterial activity in sand to induce carbonate precipitation for ground reinforcement.” Ecol. Eng. 36 (2): 112–117. https://doi.org/10.1016/j.ecoleng.2009.01.004.
Harran, R., D. Terzis, and L. Laloui. 2022. “Characterizing the deformation evolution with stress and time of biocemented sands.” J. Geotech. Geoenviron. Eng. 148 (10): 04022074. https://doi.org/10.1061/(ASCE)GT.1943-5606.0002871.
Hata, T. 2019. “A practical bio-based reversible permeability control for saturated sands.” Environ. Geotech. 8 (6): 392–400. https://doi.org/10.1680/jenge.18.00153.
Hata, T., A. C. Saracho, S. K. Haigh, J. Yoneda, and K. Yamamoto. 2020. “Microbial-induced carbonate precipitation applicability with the methane hydrate-bearing layer microbe.” J. Nat. Gas Sci. Eng. 81: 1–9. https://doi.org/10.1016/j.jngse.2020.103490.
Hata, T., D. Suetsugu, and K. Kasama. 2018. “A biomediated deterioration mitigation method for cement-treated soil.” Environ. Geotech. 7 (6): 435–444. https://doi.org/10.1680/jenge.18.00011.
He, J., V. Ivanov, and J. Chu. 2013. “Mitigation of liquefaction of saturated sand using biogas.” Geotechnique 63 (4): 267–275. https://doi.org/10.1680/geot.SIP13.P.004.
Heveran, C. M., L. Liang, A. Nagarajan, M. H. Hubler, R. Gill, J. C. Cameron, S. M. Cook, and W. V. Srubar. 2019. “Engineered ureolytic microorganisms can tailor the morphology and nanomechanical properties of microbial-precipitated calcium carbonate.” Sci. Rep. 9 (1): 1–13. https://doi.org/10.1038/s41598-019-51133-9.
Hoang, T., J. Alleman, B. Cetin, K. Ikuma, and S. G. Choi. 2019. “Sand and silty-sand soil stabilization using bacterial enzyme-induced calcite precipitation (BEICP).” Can. Geotech. J. 56 (6): 808–822. https://doi.org/10.1139/cgj-2018-0191.
Hodges, T. M., and B. N. Lingwall. 2020. “Case histories of full-scale microbial bio-cement application for surface erosion control.” In Geo-Congress 2020: Biogenetics, Geotechnical Special Publication 320, edited by E. Kavazanjian, J. P. Hambleton, R. Makhnenko, and A. S. Budge, 9–19. Reston, VA: ASCE.
Hommel, J., A. Akyel, Z. Frieling, A. J. Phillips, R. Gerlach, A. B. Cunningham, and H. Class. 2020. “A numerical model for enzymatically induced calcium carbonate precipitation.” Appl. Sci. 10 (13): 1–26. https://doi.org/10.3390/app10134538.
Hommel, J., E. Lauchnor, A. Phillips, R. Gerlach, A. B. Cunningham, R. Helmig, A. Ebigbo, and H. Class. 2015. “A revised model for microbially induced calcite precipitation: Improvements and new insights based on recent experiments.” Water Resour. Res. 51: 3695–3715. https://doi.org/10.1002/2014WR016503.
Huang, J. X., Y. H. Wang, T. A. Nguyen, C. S. Hsu, and W. Y. Hung. 2021a. “Soil improvement by microbial induced calcite precipitation and a chemical method for liquefaction mitigation.” J. Geoeng. 16 (1): 35–46. https://doi.org/10.6310/jog.202103_16(1).4.
Huang, M., K. Xu, C. Xu, G. Jin, and S. Guo. 2021b. “Micromechanical properties of biocemented shale soils analyzed using nanoindentation test.” J. Geotech. Geoenviron. Eng. 147 (12): 04021157. https://doi.org/10.1061/(asce)gt.1943-5606.0002653.
IndustryARC. 2021. Soil stabilization market—Forecast (2020–2025). Hyderabad: IndustryARC.
Jain, S., and D. N. Arnepalli. 2020. “Adhesion and deadhesion of ureolytic bacteria on sand under variable pore fluid chemistry.” J. Environ. Eng. 146 (6): 04020038. https://doi.org/10.1061/(asce)ee.1943-7870.0001708.
Jiang, N. J., and K. Soga. 2019. “Erosional behavior of gravel-sand mixtures stabilized by microbially induced calcite precipitation (MICP).” Soils Found. 59 (3): 699–709. https://doi.org/10.1016/j.sandf.2019.02.003.
Karimian, A., M. Hassanlourad, and G. R. Karimi. 2021. “Insight into the properties of surface percolated biocemented sand.” Geomicrobiol. J. 38 (2): 138–149. https://doi.org/10.1080/01490451.2020.1818147.
Kashizadeh, E., A. Mukherjee, and A. Tordesillas. 2021. “Experimental and numerical investigations on confined granular systems stabilized by bacterial cementation.” Int. J. Geomech. 21 (1): 04020244. https://doi.org/10.1061/(asce)gm.1943-5622.0001891.
Keykha, H. A., and A. Asadi. 2017. “Solar powered electro-bio-stabilization of soil with ammonium pollution prevention system.” Adv. Civ. Eng. Mater. 6 (1): 20170001. https://doi.org/10.1520/acem20170001.
Kim, D. H., N. Mahabadi, J. Jang, and L. A. van Paassen. 2020. “Assessing the kinetics and pore-scale characteristics of biological calcium carbonate precipitation in porous media using a microfluidic chip experiment.” Water Resour. Res. 56 (2): 1–19. https://doi.org/10.1029/2019WR025420.
Koliji, A., L. Vulliet, and L. Laloui. 2008. “New basis for the constitutive modelling of aggregated soils.” Acta Geotech. 3 (1): 61–69. https://doi.org/10.1007/s11440-007-0052-x.
Konstantinou, C., G. Biscontin, N. Jiang, and K. Soga. 2021. “Application of microbially induced carbonate precipitation to form bio-cemented artificial sandstone.” J. Rock Mech. Geotech. Eng. 13 (3): 579–592. https://doi.org/10.1016/j.jrmge.2021.01.010.
Landa-Marbán, D., S. Tveit, K. Kumar, and S. E. Gasda. 2021. “Practical approaches to study microbially induced calcite precipitation at the field scale.” Int. J. Greenhouse Gas Control 106: 103256. https://doi.org/10.1016/j.ijggc.2021.103256.
Lee, M., M. G. Gomez, M. El Kortbawi, and K. Ziotopoulou. 2020. “Examining the liquefaction resistance of lightly cemented sands using microbially induced calcite precipitation (MICP).” In Geo-Congress 2020: Biogeotechnics, Geotechnical Special Publication 320, edited by E. Kavazanjian, J. P. Hambleton, R. Makhnenko, and A. S. Budge, 53–64. Reston, VA: ASCE.
Lee, M. L., W. S. Ng, and Y. Tanaka. 2013. “Stress-deformation and compressibility responses of bio-mediated residual soils.” Ecol. Eng. 60: 142–149. https://doi.org/10.1016/j.ecoleng.2013.07.034.
Li, J., J. Ma, Y. Tong, E. F, and Z. Zhang. 2021a. “Study on fractal characteristics of pores of NAS reinforced by MICP under the control of electric field.” Constr. Build. Mater. 271: 121540. https://doi.org/10.1016/j.conbuildmat.2020.121540.
Li, X., C. Zhang, H. Xiao, W. Jiang, J. Qian, and Z. Li. 2021b. “Reducing compressibility of the expansive soil by microbiological-induced calcium carbonate precipitation.” Adv. Civ. Eng. 2021: 1–12. https://doi.org/10.1155/2021/8818771.
Li, Y., Z. Guo, L. Wang, Z. Ye, C. Shen, and W. Zhou. 2021c. “Interface shear behavior between MICP-treated calcareous sand and steel.” J. Mater. Civ. Eng. 33 (2): 04020455. https://doi.org/10.1061/(asce)mt.1943-5533.0003549.
Liingaard, M., A. Augustesen, and P. V. Lade. 2004. “Characterization of models for time-dependent behavior of soils.” Int. J. Geomech. 4 (3): 157–177. https://doi.org/10.1061/(ASCE)1532-3641(2004)4:3(157).
Lin, H., M. T. Suleiman, and D. G. Brown. 2020. “Investigation of pore-scale CaCO3 distributions and their effects on stiffness and permeability of sands treated by microbially induced carbonate precipitation (MICP).” Soils Found. 60 (4): 944–961. https://doi.org/10.1016/j.sandf.2020.07.003.
Lin, H., M. T. Suleiman, D. G. Brown, and E. Kavazanjian. 2016. “Mechanical behavior of sands treated by microbially induced carbonate precipitation.” J. Geotech. Geoenviron. Eng. 142 (2): 1–13. https://doi.org/10.1061/(ASCE)GT.1943-5606.0001383.
Liu, L., H. Liu, A. W. Stuedlein, T. M. Evans, and Y. Xiao. 2019. “Strength, stiffness, and microstructure characteristics of biocemented calcareous sand.” Can. Geotech. J. 56 (10): 1502–1513. https://doi.org/10.1139/cgj-2018-0007.
Lu, Y., W. Zhu, G. Ye, and F. Zhang. 2022. “A unified constitutive model for cemented/non-cemented soils under monotonic and cyclic loading.” Acta Geotech. 17: 2173–2191. https://doi.org/10.1007/s11440-021-01348-w.
Ma, G., X. He, X. Jiang, H. Liu, J. Chu, and Y. Xiao. 2021. “Strength and permeability of bentonite-assisted biocemented coarse sand.” Can. Geotech. J. 58 (7): 969–981. https://doi.org/10.1139/cgj-2020-0045.
Mahawish, A., A. Bouazza, and W. P. Gates. 2018. “Improvement of coarse sand engineering properties by microbially induced calcite precipitation.” Geomicrobiol. J. 35 (10): 887–897. https://doi.org/10.1080/01490451.2018.1488019.
Mahawish, A., A. Bouazza, and W. P. Gates. 2019. “Unconfined compressive strength and visualization of the microstructure of coarse sand subjected to different biocementation levels.” J. Geotech. Geoenviron. Eng. 145 (8): 04019033. https://doi.org/10.1061/(asce)gt.1943-5606.0002066.
Mahawish, A., A. Bouazza, and W. P. Gates. 2021. “Model tests on biogrouted granular columns in soft soil.” Can. Geotech. J. 58 (12): 1–28. https://doi.org/10.1139/cgj-2020-0361.
Maleki-kakelar, M., M. J. Azarhoosh, S. G. Senji, and A. Aghaeinejad-Meybodi. 2021. “Urease production using corn steep liquor as a low-cost nutrient source by Sporosarcina pasteurii: Biocementation and process optimization via artificial intelligence approaches.” Environ. Sci. Pollut. Res. 29 (10): 1–15.
Martinez, A., L. Huang, and M. G. Gomez. 2019. “Thermal conductivity of MICP-treated sands at varying degrees of saturation.” Geotech. Lett. 9 (1): 15–21. https://doi.org/10.1680/jgele.18.00126.
Martinez, B. C., J. T. DeJong, T. R. Ginn, B. M. Montoya, T. H. Barkouki, C. Hunt, B. Tanyu, and D. Major. 2013. “Experimental optimization of microbial-induced carbonate precipitation for soil improvement.” J. Geotech. Geoenviron. Eng. 139 (4): 587–598. https://doi.org/10.1061/(ASCE)GT.1943-5606.0000787.
Marzin, T., B. Desvages, A. Creppy, L. Lépine, A. Esnault-Filet, and H. Auradou. 2020. “Using microfluidic set-up to determine the adsorption rate of Sporosarcina pasteurii bacteria on sandstone.” Transp. Porous Media 132 (2): 283–297. https://doi.org/10.1007/s11242-020-01391-3.
Mehrabi, R., and K. Atefi-Monfared. 2021. “A coupled bio-chemo-hydro-mechanical model for bio-cementation in porous media.” Can. Geotech. J. 59 (7): 1–55. https://doi.org/10.1139/cgj-2021-0396.
Minto, J. M., R. J. Lunn, and G. El Mountassir. 2019. “Development of a reactive transport model for field-scale simulation of microbially induced carbonate precipitation.” Water Resour. Res. 55 (8): 7229–7245. https://doi.org/10.1029/2019WR025153.
Mitchell, J. K., and J. C. Santamarina. 2005. “Biological considerations in geotechnical engineering.” J. Geotech. Geoenviron. Eng. 131 (10): 1222–1233. https://doi.org/10.1061/(asce)1090-0241(2005)131:10(1222).
Montoya, B. M., J. T. DeJong, and R. W. Boulanger. 2013. “Dynamic response of liquefiable sand improved by microbial-induced calcite precipitation.” Géotechnique 63 (4): 302–312. https://doi.org/10.1680/geot.SIP13.P.019.
Montoya, B. M., S. Safavizadeh, and M. A. Gabr. 2019. “Enhancement of coal ash compressibility parameters using microbial-induced carbonate precipitation.” J. Geotech. Geoenviron. Eng. 145 (5): 04019018. https://doi.org/10.1061/(ASCE)GT.1943-5606.0002036.
Mortensen, B. M., M. J. Haber, J. T. DeJong, L. F. Caslake, and D. C. Nelson. 2011. “Effects of environmental factors on microbial induced calcium carbonate precipitation.” J. Appl. Microbiol. 111 (2): 338–349. https://doi.org/10.1111/j.1365-2672.2011.05065.x.
Moug, D. M., et al. 2022. “Field trials of microbially induced desaturation in low-plasticity silt.” J. Geotech. Geoenviron. Eng. 148 (11): 1–19. https://doi.org/10.1061/(asce)gt.1943-5606.0002890.
Mousavi, S., and M. Ghayoomi. 2021. “Liquefaction mitigation of sands with nonplastic fines via microbial-induced partial saturation.” J. Geotech. Geoenviron. Eng. 147 (2): 04020156. https://doi.org/10.1061/(asce)gt.1943-5606.0002444.
Murugan, R., G. K. Suraishkumar, A. Mukherjee, and N. K. Dhami. 2021. “Insights into the influence of cell concentration in design and development of microbially induced calcium carbonate precipitation (MICP) process.” PLoS One 16 (7): 1–19. https://doi.org/10.1371/journal.pone.0254536.
Nafisi, A., A. Khoubani, B. M. Montoya, and T. M. Evans. 2018. “The effect of grain size and shape on mechanical behavior of MICP sand I experimental study.” In Proc., 11th National Conf. in Earthquake Engineering. Los Angeles, CA: Earthquake Engineering Research Institute (EERI).
Nafisi, A., Q. Liu, and B. M. Montoya. 2021. “Effect of stress path on the shear response of bio-cemented sands.” Acta Geotech. 16 (10): 3239–3251. https://doi.org/10.1007/s11440-021-01286-7.
Nafisi, A., D. Mocelin, B. M. Montoya, and S. Underwood. 2020a. “Tensile strength of sands treated with microbially induced carbonate precipitation.” Can. Geotech. J. 57 (10): 1611–1616. https://doi.org/10.1139/cgj-2019-0230.
Nafisi, A., B. M. Montoya, and T. M. Evans. 2020b. “Shear strength envelopes of biocemented sands with varying particle size and cementation level.” J. Geotech. Geoenviron. Eng. 146 (3): 04020002. https://doi.org/10.1061/(asce)gt.1943-5606.0002201.
Nafisi, A., S. Safavizadeh, and B. M. Montoya. 2019. “Influence of microbe and enzyme-induced treatments on cemented sand shear response.” J. Geotech. Geoenviron. Eng. 145 (9): 06019008. https://doi.org/10.1061/(asce)gt.1943-5606.0002111.
Namikawa, T., S. Hiyama, Y. Ando, and T. Shibata. 2017. “Failure behavior of cement-treated soil under triaxial tension conditions.” Soils Found. 57 (5): 815–827. https://doi.org/10.1016/j.sandf.2017.08.011.
Naser, M. Z., and Q. Chen. 2021. “Extraterrestrial construction in lunar and Martian environments.” Earth Space 2021: 1200–1207.
Nature Reviews. 2011. “Microbiology by numbers.” Nat. Rev. Microbiol. 9 (9): 628. https://doi.org/10.1038/nrmicro2644.
Nething, C., M. Smirnova, J. A. D. Gröning, W. Haase, A. Stolz, and W. Sobek. 2020. “A method for 3D printing bio-cemented spatial structures using sand and urease active calcium carbonate powder.” Mater. Des. 195: 109032. https://doi.org/10.1016/j.matdes.2020.109032.
Nishimura, I., and H. Matsubara. 2021. “Coupling simulation of microbially induced carbonate precipitation and bacterial growth using reaction–diffusion and homogenisation systems.” Acta Geotech. 16 (5): 1315–1330. https://doi.org/10.1007/s11440-021-01178-w.
Nweke, C. C., and J. M. Pestana. 2018. “Modeling bio-cemented sands: A strength index for cemented sands.” In IFCEE 2018: Innovations in Ground Improvement for Soils, Pavements, and Subgrades, Geotechnical Special Publication 296, edited by A. W. Stuedlein, A. Lemnitzer, and M. T. Suleiman, 48–58. Reston, VA: ASCE.
O’Donnell, S. T., C. A. Hall, E. Kavazanjian, and B. E. Rittmann. 2019a. “Biogeochemical model for soil improvement by denitrification.” J. Geotech. Geoenviron. Eng. 145 (11): 04019091. https://doi.org/10.1061/(asce)gt.1943-5606.0002126.
O’Donnell, S. T., B. E. Rittmann, and E. Kavazanjian. 2017. “MIDP: Liquefaction mitigation via microbial denitrification as a two-stage process. I: Desaturation.” J. Geotech. Geoenviron. Eng. 143 (12): 1–11. https://doi.org/10.1061/(ASCE)GT.1943-5606.0001818.
O’Donnell, S. T., B. E. Rittmann, and E. Kavazanjian. 2019b. “Factors controlling microbially induced desaturation and precipitation (MIDP) via denitrification during continuous flow.” Geomicrobiol. J. 36 (6): 543–558. https://doi.org/10.1080/01490451.2019.1581858.
Omoregie, A. I., G. Khoshdelnezamiha, N. Senian, D. E. L. Ong, and P. M. Nissom. 2017. “Experimental optimisation of various cultural conditions on urease activity for isolated Sporosarcina pasteurii strains and evaluation of their biocement potentials.” Ecol. Eng. 109: 65–75. https://doi.org/10.1016/j.ecoleng.2017.09.012.
Park, S.-S., T.-T. Le, Z. Nong, H.-D. Moon, and D.-E. Lee. 2020. “Chemically induced calcium carbonate precipitation for improving strength of sand.” J. Mater. Civ. Eng. 32 (9): 04020238. https://doi.org/10.1061/(asce)mt.1943-5533.0003318.
Péron, H. 2013. Desiccation cracking of soils. Lausanne, Switzerland: Ecole Polytechnique Fédérale de Lausanne (EPFL).
Priya, P., K. L. Kuhlman, and N. R. Aluru. 2021. “Pore-scale modeling of electrokinetics in geomaterials.” Transp. Porous Media 137 (3): 651–666. https://doi.org/10.1007/s11242-021-01581-7.
Proto, C. J., J. T. DeJong, and D. C. Nelson. 2016. “Biomediated permeability reduction of saturated sands.” J. Geotech. Geoenviron. Eng. 142 (12): 1–11. https://doi.org/10.1061/(ASCE)GT.1943-5606.0001558.
Rahman, S., M. J. Khattak, B. Adhikari, and S. Adhikari. 2021. “Discrete element modeling of bonded soil mixtures under uniaxial compression and indirect tension test.” Transp. Geotech. 26: 100438. https://doi.org/10.1016/j.trgeo.2020.100438.
Riveros, G. A., and A. Sadrekarimi. 2020a. “Effect of microbially induced cementation on the instability and critical state behaviours of Fraser river sand.” Can. Geotech. J. 57 (12): 1870–1880. https://doi.org/10.1139/cgj-2019-0514.
Riveros, G. A., and A. Sadrekarimi. 2020b. “Liquefaction resistance of Fraser River sand improved by a microbially-induced cementation.” Soil Dyn. Earthquake Eng. 131: 106034. https://doi.org/10.1016/j.soildyn.2020.106034.
Safdar, M. U., M. Mavroulidou, M. J. Gunn, D. Purchase, I. Payne, and J. Garelick. 2021. “Electrokinetic biocementation of an organic soil.” Sustainable Chem. Pharm. 21: 100405. https://doi.org/10.1016/j.scp.2021.100405.
Saffari, R., E. Nikooee, G. Habibagahi, and M. T. van Genuchten. 2019. “Effects of biological stabilization on the water retention properties of unsaturated soils.” J. Geotech. Geoenviron. Eng. 145 (7): 04019028. https://doi.org/10.1061/(asce)gt.1943-5606.0002053.
Shariq, A. F., H. Beyenal, and I. D. Akin. 2021. “Biofilm addition improves sand strength over a wide range of saturations.” Biofilm 3: 100050. https://doi.org/10.1016/j.bioflm.2021.100050.
Sharma, M., N. Satyam, and K. R. Reddy. 2021a. “Effect of freeze–thaw cycles on engineering properties of biocemented sand under different treatment conditions.” Eng. Geol. 284: 106022. https://doi.org/10.1016/j.enggeo.2021.106022.
Sharma, M., N. Satyam, and K. R. Reddy. 2021b. “Rock-like behavior of biocemented sand treated under non-sterile environment and various treatment conditions.” J. Rock Mech. Geotech. Eng. 13 (3): 705–716. https://doi.org/10.1016/j.jrmge.2020.11.006.
Shen, Z., M. Jiang, and S. Wang. 2019. “Static and kinematic damage characterization in structured sand.” Acta Geotech. 14 (5): 1403–1421. https://doi.org/10.1007/s11440-018-0730-x.
Simatupang, M., M. Okamura, K. Hayashi, and H. Yasuhara. 2018. “Small-strain shear modulus and liquefaction resistance of sand with carbonate precipitation.” Soil Dyn. Earthquake Eng. 115: 710–718. https://doi.org/10.1016/j.soildyn.2018.09.027.
Suer, P., N. Hallberg, C. Carlsson, D. Bendz, and G. Holm. 2009. “Biogrouting compared to jet grouting: Environmental (LCA) and economical assessment.” J. Environ. Sci. Health—Part A Toxic/Hazard. Subst. Environ. Eng. 44 (4): 346–353. https://doi.org/10.1080/10934520802659679.
Tang, C. S., L. Yin, N. Jiang, C. Zhu, H. Zeng, H. Li, and B. Shi. 2020. “Factors affecting the performance of microbial-induced carbonate precipitation (MICP) treated soil: A review.” Environ. Earth Sci. 79 (5): 1–23. https://doi.org/10.1007/s12665-020-8840-9.
Tang, Y., G. Xu, J. Lian, Y. Yan, D. Fu, and W. Sun. 2019. “Research on simulation analysis method of microbial cemented sand based on discrete element method.” Adv. Mater. Sci. Eng. 2019 (1): 7173414. https://doi.org/10.1155/2019/7173414.
Terzis, D., P. Hicher, and L. Laloui. 2020a. “Direct currents stimulate carbonate mineralization for soil improvement under various chemical conditions.” Sci. Rep. 10 (1): 1–14. https://doi.org/10.1038/s41598-020-73926-z.
Terzis, D., and L. Laloui. 2018. “3-D micro-architecture and mechanical response of soil cemented via microbial-induced calcite precipitation.” Sci. Rep. 8 (1): 1–11. https://doi.org/10.1038/s41598-018-19895-w.
Terzis, D., and L. Laloui. 2019a. “A decade of progress and turning points in the understanding of bio-improved soils: A review.” Geomech. Energy Environ. 19: 100116. https://doi.org/10.1016/j.gete.2019.03.001.
Terzis, D., and L. Laloui. 2019b. “Cell-free soil bio-cementation with strength, dilatancy and fabric characterization.” Acta Geotech. 14: 639–656. https://doi.org/10.1007/s11440-019-00764-3.
Terzis, D., and L. Laloui. 2021. Method and system for producing a carbonate-containing species-rich, nitrogen-containing species-free solution. Geneva: World Intellectual Property Organization.
Terzis, D., L. Laloui, S. Dornberger, and R. Harran. 2020b. “A full-scale application of slope stabilization via calcite bio-mineralization followed by long-term GIS surveillance.” In Geo-Congress 2020: Biogeotechnics, Geotechnical Special Publication 320, edited by E. Kavazanjian, J. P. Hambleton, R. Makhnenko, and A. S. Budge, 65–73. Reston, VA: ASCE.
Tian, Z., X. Tang, J. Li, Z. Xiu, and Z. Xue. 2021. “Improving settlement and reinforcement uniformity of marine clay in electro-osmotic consolidation using microbially induced carbonate precipitation.” Bull. Eng. Geol. Environ. 80: 6457–6471. https://doi.org/10.1007/s10064-021-02305-3.
Tiwari, N., N. Satyam, and M. Sharma. 2021. “Micro-mechanical performance evaluation of expansive soil biotreated with indigenous bacteria using MICP method.” Sci. Rep. 11 (1): 1–12. https://doi.org/10.1038/s41598-020-79139-8.
USEPA. 2013. Aquatic life ambient water quality criteria for ammonia - Freshwater 2013, 1–255. Washington, DC: USEPA.
Van Paassen, L. A. 2011. “Bio-mediated ground improvement: From laboratory experiment to pilot applications.” In Geo-Frontiers, Geotechnical Special Publication 211, edited by J. Han and D. E. Alzamora, 4099–4107. Reston, VA: ASCE.
Van Paassen, L. A., R. Ghose, T. J. M. van der Linden, W. R. L. van der Star, and M. C. M. van Loosdrecht. 2010. “Quantifying biomediated ground improvement by ureolysis: Large-scale BioGrout experiment.” J. Geotech. Geoenviron. Eng. 136 (12): 1721–1728. https://doi.org/10.1061/(ASCE)GT.1943-5606.0000382.
Van Paassen, L. A., M. P. Harkes, G. A. Van Zwieten, W. H. Van Der Zon, W. R. L. Van Der Star, and M. C. M. Van Loosdrecht. 2009. “Scale up of BioGrout: A biological ground reinforcement method.” In Vol. 3 of Proc., 17th Int. Conf. on Soil Mechanics and Geotechnical Engineering: The Academia and Practice of Geotechnical Engineering, 2328–2333. London: IOP Press.
Venuleo, S., L. Laloui, D. Terzis, T. Hueckel, and M. Hassan. 2016. “Microbially induced calcite precipitation effect on soil thermal conductivity.” Géotech. Lett. 6 (1): 39–44. https://doi.org/10.1680/jgele.15.00125.
Wang, H., and Z. Yin. 2021. “Unconfined compressive strength of bio-cemented sand: State-of-the-art review and MEP-MC-based model development.” J. Cleaner Prod. 315: 128205. https://doi.org/10.1016/j.jclepro.2021.128205.
Wang, J., P. Fan, M. Wang, L. Dong, L. Ma, and L. Gao. 2020a. “Experimental study on creep properties of coral sand.” Can. Geotech. J. 57: 1854–1869. https://doi.org/10.11779/CJGE202011018.
Wang, L., J. Chu, S. Wu, and H. Wang. 2021a. “Stress–dilatancy behavior of cemented sand: Comparison between bonding provided by cement and biocement.” Acta Geotech. 4: 1–16. https://doi.org/10.1007/s11440-021-01146-4.
Wang, L., Y. Gao, J. He, Y. Gao, and L. A. van Paassen. 2021b. “Effect of biogenic gas formation through microbial induced desaturation and precipitation on the static response of sands with varied relative density.” J. Geotech. Geoenviron. Eng. 147 (8): 04021071. https://doi.org/10.1061/(asce)gt.1943-5606.0002578.
Wang, L., X. Jiang, X. He, J. Chu, Y. Xiao, H. Liu, and E. K. H. Salje. 2021c. “Crackling noise and bio-cementation.” Eng. Fract. Mech. 247: 107675. https://doi.org/10.1016/j.engfracmech.2021.107675.
Wang, L., L. Van Paassen, Y. Gao, J. He, Y. Gao, and D. Kim. 2021d. “Laboratory tests on mitigation of soil liquefaction using microbial induced desaturation and precipitation.” Geotech. Test. J. 44 (2): 520–534. https://doi.org/10.1520/GTJ20190432.
Wang, X., and U. Nackenhorst. 2020. “A coupled bio-chemo-hydraulic model to predict porosity and permeability reduction during microbially induced calcite precipitation.” Adv. Water Resour. 139: 103563. https://doi.org/10.1016/j.advwatres.2020.103563.
Wang, Y., K. Soga, J. T. DeJong, and A. J. Kabla. 2019a. “A microfluidic chip and its use in characterising the particle-scale behaviour of microbial-induced calcium carbonate precipitation (MICP).” Geotechnique 69 (12): 1086–1094. https://doi.org/10.1680/jgeot.18.P.031.
Wang, Y., K. Soga, J. T. DeJong, and A. J. Kabla. 2019b. “Microscale visualization of microbial-induced calcium carbonate precipitation processes.” J. Geotech. Geoenviron. Eng. 145 (9): 04019045. https://doi.org/10.1061/(asce)gt.1943-5606.0002079.
Wang, Y., K. Soga, J. T. DeJong, and A. J. Kabla. 2021e. “Effects of bacterial density on growth rate and characteristics of microbial-induced CaCO3 precipitates: Particle-scale experimental study.” J. Geotech. Geoenviron. Eng. 147 (6): 1–13. https://doi.org/10.1061/(asce)gt.1943-5606.0002509.
Wang, Z., N. Zhang, J. Ding, Q. Li, and J. Xu. 2020b. “Thermal conductivity of sands treated with microbially induced calcite precipitation (MICP) and model prediction.” Int. J. Heat Mass Transfer 147: 118899. https://doi.org/10.1016/j.ijheatmasstransfer.2019.118899.
Weinhardt, F., H. Class, S. Vahid Dastjerdi, N. Karadimitriou, D. Lee, and H. Steeb. 2021. “Experimental methods and imaging for enzymatically induced calcite precipitation in a microfluidic cell.” Water Resour. Res. 57 (3): 1–11. https://doi.org/10.1029/2020WR029361.
Wu, C., B. A. Jang, and H. S. Jang. 2019. “Mechanical behaviour of bio-grouted coarse-grained soil: Discrete element modelling.” J. Eng. Geol. 29 (4): 383–391.
Wu, H., W. Wu, W. Liang, F. Dai, H. Liu, and Y. Xiao. 2023. “3D DEM modeling of biocemented sand with fines as cementing agents.” Int. J. Numer. Anal. Methods Geomech. 47 (2): 212–240. https://doi.org/10.1002/nag.3466.
Wu, S., B. Li, and J. Chu. 2021. “Stress-dilatancy behavior of MICP-treated sand.” Int. J. Geomech. 21 (3): 04020264. https://doi.org/10.1061/(asce)gm.1943-5622.0001923.
Xiao, P., H. Liu, A. W. Stuedlein, T. M. Evans, and Y. Xiao. 2019a. “Effect of relative density and biocementation on cyclic response of calcareous sand.” Can. Geotech. J. 56 (12): 1849–1862. https://doi.org/10.1139/cgj-2018-0573.
Xiao, P., H. Liu, Y. Xiao, A. W. Stuedlein, and T. M. Evans. 2018. “Liquefaction resistance of bio-cemented calcareous sand.” Soil Dyn. Earthquake Eng. 107: 9–19. https://doi.org/10.1016/j.soildyn.2018.01.008.
Xiao, Y., H. Chen, A. W. Stuedlein, T. M. Evans, J. Chu, L. Cheng, N. Jiang, H. Lin, H. Liu, and H. M. Aboel-Naga. 2020a. “Restraint of particle breakage by biotreatment method.” J. Geotech. Geoenviron. Eng. 146 (11): 04020123. https://doi.org/10.1061/(asce)gt.1943-5606.0002384.
Xiao, Y., X. He, T. M. Evans, A. W. Stuedlein, and H. Liu. 2019b. “Unconfined compressive and splitting tensile strength of basalt fiber–reinforced biocemented sand.” J. Geotech. Geoenviron. Eng. 145 (9): 04019048. https://doi.org/10.1061/(asce)gt.1943-5606.0002108.
Xiao, Y., X. He, A. W. Stuedlein, J. Chu, T. Matthew Evans, and L. A. van Paassen. 2022a. “Crystal growth of MICP through microfluidic chip tests.” J. Geotech. Geoenviron. Eng. 148 (5): 1–9. https://doi.org/10.1061/(asce)gt.1943-5606.0002756.
Xiao, Y., X. He, W. Wu, A. W. Stuedlein, T. M. Evans, J. Chu, H. Liu, L. A. van Paassen, and H. Wu. 2021a. “Kinetic biomineralization through microfluidic chip tests.” Acta Geotech. 16: 3229–3237. https://doi.org/10.1007/s11440-021-01205-w.
Xiao, Y., X. He, M. Zaman, G. Ma, and C. Zhao. 2022b. “Review of strength improvements of biocemented soils.” Int. J. Geomech. 22 (11): 1–23. https://doi.org/10.1061/(asce)gm.1943-5622.0002565.
Xiao, Y., A. W. Stuedlein, Z. Pan, H. Liu, T. Matthew Evans, X. He, H. Lin, J. Chu, and L. A. van Paassen. 2020b. “Toe-bearing capacity of precast concrete piles through biogrouting improvement.” J. Geotech. Geoenviron. Eng. 146 (12): 06020026. https://doi.org/10.1061/(asce)gt.1943-5606.0002404.
Xiao, Y., A. W. Stuedlein, J. Ran, T. M. Evans, L. Cheng, H. Liu, L. A. van Paassen, and J. Chu. 2019c. “Effect of particle shape on strength and stiffness of biocemented glass beads.” J. Geotech. Geoenviron. Eng. 145 (11): 06019016. https://doi.org/10.1061/(asce)gt.1943-5606.0002165.
Xiao, Y., Y. Tang, G. Ma, J. S. McCartney, and J. Chu. 2021b. “Thermal conductivity of biocemented graded sands.” J. Geotech. Geoenviron. Eng. 147 (10): 04021106. https://doi.org/10.1061/(asce)gt.1943-5606.0002621.
Xiao, Y., Y. Wang, S. Wang, T. M. Evans, A. W. Stuedlein, J. Chu, C. Zhao, H. Wu, and H. Liu. 2021c. “Homogeneity and mechanical behaviors of sands improved by a temperature-controlled one-phase MICP method.” Acta Geotech. 16 (5): 1417–1427. https://doi.org/10.1007/s11440-020-01122-4.
Xiao, Y., W. Xiao, G. Ma, X. He, H. Wu, and J. Shi. 2022c. “Mechanical performance of biotreated sandy road bases.” J. Perform. Constr. Facil 36 (1): 1–11. https://doi.org/10.1061/(asce)cf.1943-5509.0001671.
Xiao, Y., W. Xiao, H. Wu, Y. Liu, and H. Liu. 2023. “Fracture of interparticle MICP bonds under compression.” Int. J. Geomech. 23 (3): 1–12. https://doi.org/10.1061/ijgnai.gmeng-8282.
Xiao, Y., Z. Zhang, A. W. Stuedlein, and T. M. Evans. 2021d. “Liquefaction modeling for biocemented calcareous sand.” J. Geotech. Geoenviron. Eng. 147 (12): 04021149. https://doi.org/10.1061/(asce)gt.1943-5606.0002666.
Xiao, Y., C. Zhao, Y. Sun, S. Wang, H. Wu, H. Chen, and H. Liu. 2020c. “Compression behavior of MICP-treated sand with various gradations.” Acta Geotech. 2: 1–10. https://doi.org/10.1007/s11440-020-01116-2.
Xiao, Y., W. Zhou, J. Shi, H. Lu, and Z. Zhang. 2022d. “Erosion of biotreated field-scale slopes under rainfalls.” J. Perform. Constr. Facil 36 (3): 1–10. https://doi.org/10.1061/(asce)cf.1943-5509.0001732.
Xu, K., M. Huang, J. Zhen, C. Xu, and M. Cui. 2023. “Field implementation of enzyme-induced carbonate precipitation technology for reinforcing a bedding layer beneath an underground cable duct.” J. Rock Mech. Geotech. Eng. 15 (4): 1011–1022. https://doi.org/10.1016/j.jrmge.2022.06.012.
Xu, X., H. Guo, M. Li, and X. Deng. 2021. “Bio-cementation improvement via CaCO3 cementation pattern and crystal polymorph: A review.” Constr. Build. Mater. 297: 123478. https://doi.org/10.1016/j.conbuildmat.2021.123478.
Yang, P., E. Kavazanjian, and N. Neithalath. 2019. “Particle-scale mechanisms in undrained triaxial compression of biocemented sands: Insights from 3D DEM simulations with flexible boundary.” Int. J. Geomech. 19 (4): 04019009. https://doi.org/10.1061/(asce)gm.1943-5622.0001346.
Yao, D., J. Wu, G. Wang, P. Wang, J. J. Zheng, J. Yan, L. Xu, and Y. Yan. 2021. “Effect of wool fiber addition on the reinforcement of loose sands by microbially induced carbonate precipitation (MICP): Mechanical property and underlying mechanism.” Acta Geotech. 16 (5): 1401–1416. https://doi.org/10.1007/s11440-020-01112-6.
Zamani, A., and B. M. Montoya. 2019. “Undrained cyclic response of silty sands improved by microbial induced calcium carbonate precipitation.” Soil Dyn. Earthquake Eng. 120: 436–448. https://doi.org/10.1016/j.soildyn.2019.01.010.
Zambare, N. M., N. Y. Naser, R. Gerlach, and C. B. Chang. 2020. “Mineralogy of microbially induced calcium carbonate precipitates formed using single cell drop-based microfluidics.” Sci. Rep. 10 (1): 1–11. https://doi.org/10.1038/s41598-020-73870-y.
Zehner, J., A. Røyne, and P. Sikorski. 2021. “A sample cell for the study of enzyme-induced carbonate precipitation at the grain-scale and its implications for biocementation.” Sci. Rep. 11 (1): 13675. https://doi.org/10.1038/s41598-021-92235-7.
Zeng, C., Y. Veenis, C. A. Hall, E. S. Young, W. R. L. van der Star, J. Zheng, and L. A. van Paassen. 2021. “Experimental and numerical analysis of a field trial application of microbially induced calcite precipitation for ground stabilization.” J. Geotech. Geoenviron. Eng. 147 (7): 05021003. https://doi.org/10.1061/(asce)gt.1943-5606.0002545.
Zhang, J., C. Li, S. Wang, and S. Bai. 2021a. “Three-dimensional reconstruction of biomineralized sand and particle-flow-code numerical simulation of penetration resistance characteristics of biomineralization crust.” Int. J. Geomech. 21 (5): 04021048. https://doi.org/10.1061/(asce)gm.1943-5622.0002001.
Zhang, X., Y. Chen, H. Liu, Z. Zhang, and X. Ding. 2020. “Performance evaluation of a MICP-treated calcareous sandy foundation using shake table tests.” Soil Dyn. Earthquake Eng. 129: 105959. https://doi.org/10.1016/j.soildyn.2019.105959.
Zhang, Y., Q. Tang, P. Shi, and T. Katsumi. 2021b. “Influence of bio-clogging on permeability characteristics of soil.” Geotext. Geomembr. 49 (3): 707–721. https://doi.org/10.1016/j.geotexmem.2020.11.010.
Zhang, Z., L. Li, and Z. Xu. 2021c. “A thermodynamics-based hyperelastic-plastic coupled model unified for unbonded and bonded soils.” Int. J. Plast. 137: 102902. https://doi.org/10.1016/j.ijplas.2020.102902.
Zhao, Y., Z. Xiao, C. Fan, W. Shen, Q. Wang, and P. Liu. 2020. “Comparative mechanical behaviors of four fiber-reinforced sand cemented by microbially induced carbonate precipitation.” Bull. Eng. Geol. Environ. 79 (6): 3075–3086. https://doi.org/10.1007/s10064-020-01756-4.
Zhao, Y., P. Zhang, H. Fang, C. Guo, B. Zhang, and F. Wang. 2021. “Bentonite-assisted microbial-induced carbonate precipitation for coarse soil improvement.” Bull. Eng. Geol. Environ. 80 (7): 5623–5632. https://doi.org/10.1007/s10064-021-02302-6.
Zhu, C., M. Vail, C. S. Tang, L. Anderson, M. Moroski, and M. T. Montalbo-Lomboy. 2019. “Desiccation cracking behavior of MICP-treated bentonite.” Geosciences (Switzerland) 9 (9): 385. https://doi.org/10.3390/geosciences9090385.
Information & Authors
Information
Published In
Copyright
This work is made available under the terms of the Creative Commons Attribution 4.0 International license, https://creativecommons.org/licenses/by/4.0/.
History
Received: Sep 29, 2022
Accepted: Apr 21, 2023
Published online: Jul 10, 2023
Published in print: Sep 1, 2023
Discussion open until: Dec 10, 2023
ASCE Technical Topics:
- Business management
- Carbonation
- Cement
- Chemical processes
- Chemistry
- Climates
- Concrete
- Engineering materials (by type)
- Environmental engineering
- Geomechanics
- Geotechnical engineering
- Materials engineering
- Meteorology
- Microbes
- Mitigation and remediation
- Organisms
- Pollution
- Practice and Profession
- Precipitation
- Soil liquefaction
- Soil mechanics
- Soil pollution
- Soil properties
- Soil treatment
Authors
Metrics & Citations
Metrics
Citations
Download citation
If you have the appropriate software installed, you can download article citation data to the citation manager of your choice. Simply select your manager software from the list below and click Download.